Switch from translation to RNA replication in a positive-stranded RNA virus
- PMID: 9694795
- PMCID: PMC317040
- DOI: 10.1101/gad.12.15.2293
Switch from translation to RNA replication in a positive-stranded RNA virus
Abstract
In positive-stranded viruses, the genomic RNA serves as a template for both translation and RNA replication. Using poliovirus as a model, we examined the interaction between these two processes. We show that the RNA polymerase is unable to replicate RNA templates undergoing translation. We discovered that an RNA structure at the 5' end of the viral genome, next to the internal ribosomal entry site, carries signals that control both viral translation and RNA synthesis. The interaction of this RNA structure with the cellular factor PCBP up-regulates viral translation, while the binding of the viral protein 3CD represses translation and promotes negative-strand RNA synthesis. We propose that the interaction of 3CD with this RNA structure controls whether the genomic RNA is used for translation or RNA replication.
Figures
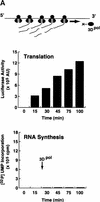
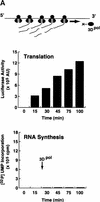
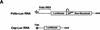

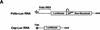

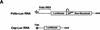

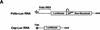

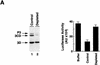
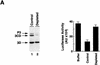
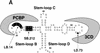
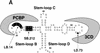
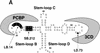
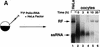
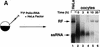
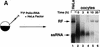
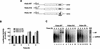
Similar articles
-
Interactions of viral protein 3CD and poly(rC) binding protein with the 5' untranslated region of the poliovirus genome.J Virol. 2000 Mar;74(5):2219-26. doi: 10.1128/jvi.74.5.2219-2226.2000. J Virol. 2000. PMID: 10666252 Free PMC article.
-
Translation of polioviral mRNA is inhibited by cleavage of polypyrimidine tract-binding proteins executed by polioviral 3C(pro).J Virol. 2002 Mar;76(5):2529-42. doi: 10.1128/jvi.76.5.2529-2542.2002. J Virol. 2002. PMID: 11836431 Free PMC article.
-
Cellular protein modification by poliovirus: the two faces of poly(rC)-binding protein.J Virol. 2007 Sep;81(17):8919-32. doi: 10.1128/JVI.01013-07. Epub 2007 Jun 20. J Virol. 2007. PMID: 17581994 Free PMC article.
-
Mechanistic intersections between picornavirus translation and RNA replication.Adv Virus Res. 2011;80:1-24. doi: 10.1016/B978-0-12-385987-7.00001-4. Adv Virus Res. 2011. PMID: 21762819 Review.
-
Molecular biology and cell-free synthesis of poliovirus.Biologicals. 1993 Dec;21(4):349-56. doi: 10.1006/biol.1993.1095. Biologicals. 1993. PMID: 8024750 Review.
Cited by
-
Poliovirus RNA replication requires genome circularization through a protein-protein bridge.Mol Cell. 2001 Mar;7(3):581-91. doi: 10.1016/s1097-2765(01)00205-2. Mol Cell. 2001. PMID: 11463383 Free PMC article.
-
Selective repression of translation by the brome mosaic virus 1a RNA replication protein.J Virol. 2007 Feb;81(4):1601-9. doi: 10.1128/JVI.01991-06. Epub 2006 Nov 15. J Virol. 2007. PMID: 17108036 Free PMC article.
-
Targeting a KH-domain protein with RNA decoys.RNA. 2002 Sep;8(9):1160-73. doi: 10.1017/s135583820202808x. RNA. 2002. PMID: 12358435 Free PMC article.
-
A zinc finger-containing papain-like protease couples subgenomic mRNA synthesis to genome translation in a positive-stranded RNA virus.Proc Natl Acad Sci U S A. 2001 Feb 13;98(4):1889-94. doi: 10.1073/pnas.98.4.1889. Epub 2001 Feb 6. Proc Natl Acad Sci U S A. 2001. PMID: 11172046 Free PMC article.
-
Translational control in positive strand RNA plant viruses.Virology. 2006 Jan 5;344(1):185-97. doi: 10.1016/j.virol.2005.09.031. Virology. 2006. PMID: 16364749 Free PMC article. Review.
References
-
- Andino R, Rieckhof GE, Baltimore D. A functional ribonucleoprotein complex forms around the 5′ end of poliovirus RNA. Cell. 1990a;63:369–380. - PubMed
Publication types
MeSH terms
Substances
Grants and funding
LinkOut - more resources
Full Text Sources
Other Literature Sources