A multi-organ chip with matured tissue niches linked by vascular flow
- PMID: 35478225
- PMCID: PMC9250010
- DOI: 10.1038/s41551-022-00882-6
A multi-organ chip with matured tissue niches linked by vascular flow
Abstract
Engineered tissues can be used to model human pathophysiology and test the efficacy and safety of drugs. Yet, to model whole-body physiology and systemic diseases, engineered tissues with preserved phenotypes need to physiologically communicate. Here we report the development and applicability of a tissue-chip system in which matured human heart, liver, bone and skin tissue niches are linked by recirculating vascular flow to allow for the recapitulation of interdependent organ functions. Each tissue is cultured in its own optimized environment and is separated from the common vascular flow by a selectively permeable endothelial barrier. The interlinked tissues maintained their molecular, structural and functional phenotypes over 4 weeks of culture, recapitulated the pharmacokinetic and pharmacodynamic profiles of doxorubicin in humans, allowed for the identification of early miRNA biomarkers of cardiotoxicity, and increased the predictive values of clinically observed miRNA responses relative to tissues cultured in isolation and to fluidically interlinked tissues in the absence of endothelial barriers. Vascularly linked and phenotypically stable matured human tissues may facilitate the clinical applicability of tissue chips.
© 2022. The Author(s), under exclusive licence to Springer Nature Limited.
Figures
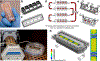
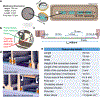
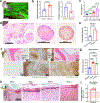
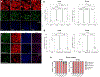
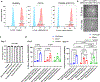
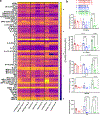
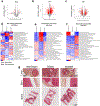
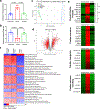
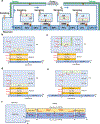
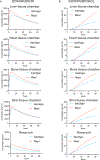
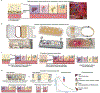
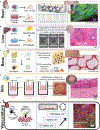
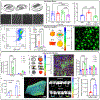
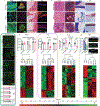
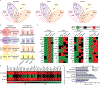
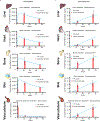
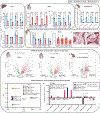
Comment in
-
Modeling multi-organ systems on a chip.Nat Methods. 2022 Jun;19(6):641. doi: 10.1038/s41592-022-01533-z. Nat Methods. 2022. PMID: 35689023 No abstract available.
-
Towards establishing human body-on-a-chip systems.Stem Cell Res Ther. 2022 Aug 20;13(1):431. doi: 10.1186/s13287-022-03130-5. Stem Cell Res Ther. 2022. PMID: 35987699 Free PMC article.
Similar articles
-
Robotic fluidic coupling and interrogation of multiple vascularized organ chips.Nat Biomed Eng. 2020 Apr;4(4):407-420. doi: 10.1038/s41551-019-0497-x. Epub 2020 Jan 27. Nat Biomed Eng. 2020. PMID: 31988458 Free PMC article.
-
A multi-organ chip co-culture of neurospheres and liver equivalents for long-term substance testing.J Biotechnol. 2015 Jul 10;205:36-46. doi: 10.1016/j.jbiotec.2015.02.002. Epub 2015 Feb 9. J Biotechnol. 2015. PMID: 25678136
-
Integrated human organ-on-a-chip model for predictive studies of anti-tumor drug efficacy and cardiac safety.Lab Chip. 2020 Nov 24;20(23):4357-4372. doi: 10.1039/d0lc00424c. Lab Chip. 2020. PMID: 32955072 Free PMC article.
-
Organ-on-a-Chip Technology for Reproducing Multiorgan Physiology.Adv Healthc Mater. 2018 Jan;7(2). doi: 10.1002/adhm.201700419. Epub 2017 Sep 25. Adv Healthc Mater. 2018. PMID: 28945001 Review.
-
Physiologically Based Pharmacokinetic and Pharmacodynamic Analysis Enabled by Microfluidically Linked Organs-on-Chips.Annu Rev Pharmacol Toxicol. 2018 Jan 6;58:37-64. doi: 10.1146/annurev-pharmtox-010716-104748. Annu Rev Pharmacol Toxicol. 2018. PMID: 29309256 Review.
Cited by
-
The mouse multi-organ proteome from infancy to adulthood.Nat Commun. 2024 Jul 9;15(1):5752. doi: 10.1038/s41467-024-50183-6. Nat Commun. 2024. PMID: 38982135 Free PMC article.
-
The Role of Biophysical Factors in Organ Development: Insights from Current Organoid Models.Bioengineering (Basel). 2024 Jun 18;11(6):619. doi: 10.3390/bioengineering11060619. Bioengineering (Basel). 2024. PMID: 38927855 Free PMC article. Review.
-
Patient-Derived Microphysiological Systems for Precision Medicine.Adv Healthc Mater. 2024 Mar;13(7):e2303161. doi: 10.1002/adhm.202303161. Epub 2023 Dec 10. Adv Healthc Mater. 2024. PMID: 38010253 Free PMC article. Review.
-
(3D) Bioprinting-Next Dimension of the Pharmaceutical Sector.Pharmaceuticals (Basel). 2024 Jun 17;17(6):797. doi: 10.3390/ph17060797. Pharmaceuticals (Basel). 2024. PMID: 38931464 Free PMC article. Review.
-
Integrating mechanical cues with engineered platforms to explore cardiopulmonary development and disease.iScience. 2023 Nov 15;26(12):108472. doi: 10.1016/j.isci.2023.108472. eCollection 2023 Dec 15. iScience. 2023. PMID: 38077130 Free PMC article. Review.
References
Publication types
MeSH terms
Substances
Grants and funding
LinkOut - more resources
Full Text Sources
Other Literature Sources