Receptor tyrosine kinases regulate signal transduction through a liquid-liquid phase separated state
- PMID: 35231400
- PMCID: PMC8937303
- DOI: 10.1016/j.molcel.2022.02.005
Receptor tyrosine kinases regulate signal transduction through a liquid-liquid phase separated state
Abstract
The recruitment of signaling proteins into activated receptor tyrosine kinases (RTKs) to produce rapid, high-fidelity downstream response is exposed to the ambiguity of random diffusion to the target site. Liquid-liquid phase separation (LLPS) overcomes this by providing elevated, localized concentrations of the required proteins while impeding competitor ligands. Here, we show a subset of phosphorylation-dependent RTK-mediated LLPS states. We then investigate the formation of phase-separated droplets comprising a ternary complex including the RTK, (FGFR2); the phosphatase, SHP2; and the phospholipase, PLCγ1, which assembles in response to receptor phosphorylation. SHP2 and activated PLCγ1 interact through their tandem SH2 domains via a previously undescribed interface. The complex of FGFR2 and SHP2 combines kinase and phosphatase activities to control the phosphorylation state of the assembly while providing a scaffold for active PLCγ1 to facilitate access to its plasma membrane substrate. Thus, LLPS modulates RTK signaling, with potential consequences for therapeutic intervention.
Keywords: FGFR2; Liquid-liquid phase separation (LLPS); Plcγ1; Receptor tyrosine kinases (RTKs); Shp2; kinase activity; phosphatase activity; phospholipase activity.
Copyright © 2022 The Author(s). Published by Elsevier Inc. All rights reserved.
Conflict of interest statement
Declaration of interests The authors declare no competing interests.
Figures
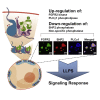
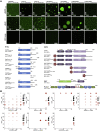
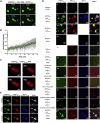
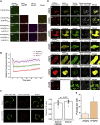
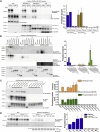
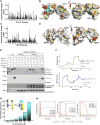
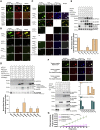
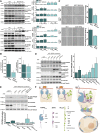
Comment in
-
In unity, there is strength: Phase separation controls receptor tyrosine kinase signal transduction.Mol Cell. 2022 Mar 17;82(6):1081-1083. doi: 10.1016/j.molcel.2022.03.003. Mol Cell. 2022. PMID: 35303480
Similar articles
-
TNF-alpha induces tyrosine phosphorylation and recruitment of the Src homology protein-tyrosine phosphatase 2 to the gp130 signal-transducing subunit of the IL-6 receptor complex.J Immunol. 2003 Jul 1;171(1):257-66. doi: 10.4049/jimmunol.171.1.257. J Immunol. 2003. PMID: 12817006
-
Activation of the protein tyrosine phosphatase SHP2 via the interleukin-6 signal transducing receptor protein gp130 requires tyrosine kinase Jak1 and limits acute-phase protein expression.Biochem J. 1998 Nov 1;335 ( Pt 3)(Pt 3):557-65. doi: 10.1042/bj3350557. Biochem J. 1998. PMID: 9794795 Free PMC article.
-
In unity, there is strength: Phase separation controls receptor tyrosine kinase signal transduction.Mol Cell. 2022 Mar 17;82(6):1081-1083. doi: 10.1016/j.molcel.2022.03.003. Mol Cell. 2022. PMID: 35303480
-
A comprehensive review of SHP2 and its role in cancer.Cell Oncol (Dordr). 2022 Oct;45(5):729-753. doi: 10.1007/s13402-022-00698-1. Epub 2022 Sep 6. Cell Oncol (Dordr). 2022. PMID: 36066752 Review.
-
The Landscape of Protein Tyrosine Phosphatase (Shp2) and Cancer.Curr Pharm Des. 2018;24(32):3767-3777. doi: 10.2174/1381612824666181106100837. Curr Pharm Des. 2018. PMID: 30398108 Review.
Cited by
-
Emerging roles of liquid-liquid phase separation in liver innate immunity.Cell Commun Signal. 2024 Sep 3;22(1):430. doi: 10.1186/s12964-024-01787-4. Cell Commun Signal. 2024. PMID: 39227829 Free PMC article. Review.
-
The Tyrosine Phosphatase SHP2: A New Target for Insulin Resistance?Biomedicines. 2022 Aug 31;10(9):2139. doi: 10.3390/biomedicines10092139. Biomedicines. 2022. PMID: 36140242 Free PMC article. Review.
-
Active RNA synthesis patterns nuclear condensates.bioRxiv [Preprint]. 2024 Oct 13:2024.10.12.614958. doi: 10.1101/2024.10.12.614958. bioRxiv. 2024. PMID: 39498261 Free PMC article. Preprint.
-
Enzyme Is the Name-Adapter Is the Game.Cells. 2024 Jul 25;13(15):1249. doi: 10.3390/cells13151249. Cells. 2024. PMID: 39120280 Free PMC article. Review.
-
Therapeutic advances of targeting receptor tyrosine kinases in cancer.Signal Transduct Target Ther. 2024 Aug 14;9(1):201. doi: 10.1038/s41392-024-01899-w. Signal Transduct Target Ther. 2024. PMID: 39138146 Free PMC article. Review.
References
-
- Agazie Y.M., Hayman M.J. Development of an efficient “substrate-trapping” mutant of Src homology phosphotyrosine phosphatase 2 and identification of the epidermal growth factor receptor, Gab1, and three other proteins as target substrates. J. Biol. Chem. 2003;278:13952–13958. - PubMed
Publication types
MeSH terms
Substances
Grants and funding
LinkOut - more resources
Full Text Sources
Molecular Biology Databases
Research Materials
Miscellaneous