The Jun-dependent axon regeneration gene program: Jun promotes regeneration over plasticity
- PMID: 34718572
- PMCID: PMC9029231
- DOI: 10.1093/hmg/ddab315
The Jun-dependent axon regeneration gene program: Jun promotes regeneration over plasticity
Erratum in
-
Erratum to: The Jun-dependent axon regeneration gene program: Jun promotes regeneration over plasticity.Hum Mol Genet. 2022 Apr 22;31(8):1356. doi: 10.1093/hmg/ddac044. Hum Mol Genet. 2022. PMID: 35166771 Free PMC article. No abstract available.
Abstract
The regeneration-associated gene (RAG) expression program is activated in injured peripheral neurons after axotomy and enables long-distance axon re-growth. Over 1000 genes are regulated, and many transcription factors are upregulated or activated as part of this response. However, a detailed picture of how RAG expression is regulated is lacking. In particular, the transcriptional targets and specific functions of the various transcription factors are unclear. Jun was the first-regeneration-associated transcription factor identified and the first shown to be functionally important. Here we fully define the role of Jun in the RAG expression program in regenerating facial motor neurons. At 1, 4 and 14 days after axotomy, Jun upregulates 11, 23 and 44% of the RAG program, respectively. Jun functions relevant to regeneration include cytoskeleton production, metabolic functions and cell activation, and the downregulation of neurotransmission machinery. In silico analysis of promoter regions of Jun targets identifies stronger over-representation of AP1-like sites than CRE-like sites, although CRE sites were also over-represented in regions flanking AP1 sites. Strikingly, in motor neurons lacking Jun, an alternative SRF-dependent gene expression program is initiated after axotomy. The promoters of these newly expressed genes exhibit over-representation of CRE sites in regions near to SRF target sites. This alternative gene expression program includes plasticity-associated transcription factors and leads to an aberrant early increase in synapse density on motor neurons. Jun thus has the important function in the early phase after axotomy of pushing the injured neuron away from a plasticity response and towards a regenerative phenotype.
© The Author(s) 2021. Published by Oxford University Press.
Figures
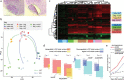
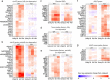
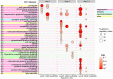
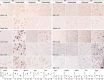
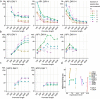
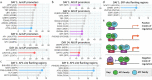
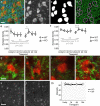
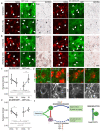
Similar articles
-
Axonal injury and peripheral nerve grafting in the thalamus and cerebellum of the adult rat: upregulation of c-jun and correlation with regenerative potential.Eur J Neurosci. 1998 Aug;10(8):2644-56. doi: 10.1046/j.1460-9568.1998.00282.x. Eur J Neurosci. 1998. PMID: 9767394
-
The AP-1 transcription factor c-Jun is required for efficient axonal regeneration.Neuron. 2004 Jul 8;43(1):57-67. doi: 10.1016/j.neuron.2004.06.005. Neuron. 2004. PMID: 15233917
-
Neuronal c-Jun is required for successful axonal regeneration, but the effects of phosphorylation of its N-terminus are moderate.J Neurochem. 2012 May;121(4):607-18. doi: 10.1111/j.1471-4159.2012.07706.x. Epub 2012 Mar 21. J Neurochem. 2012. PMID: 22372722 Free PMC article.
-
Turning on the machine: genetic control of axon regeneration by c-Jun.Neuron. 2004 Jul 8;43(1):1-2. doi: 10.1016/j.neuron.2004.06.020. Neuron. 2004. PMID: 15233911 Review.
-
Cell death and axon regeneration of Purkinje cells after axotomy: challenges of classical hypotheses of axon regeneration.Brain Res Brain Res Rev. 2005 Sep;49(2):300-16. doi: 10.1016/j.brainresrev.2004.11.007. Epub 2005 Mar 2. Brain Res Brain Res Rev. 2005. PMID: 16111558 Review.
Cited by
-
Navigating the Gene Co-Expression Network and Drug Repurposing Opportunities for Brain Disorders Associated with Neurocognitive Impairment.Brain Sci. 2023 Nov 7;13(11):1564. doi: 10.3390/brainsci13111564. Brain Sci. 2023. PMID: 38002524 Free PMC article.
-
Neuron-specific RNA-sequencing reveals different responses in peripheral neurons after nerve injury.Elife. 2024 May 14;12:RP91316. doi: 10.7554/eLife.91316. Elife. 2024. PMID: 38742628 Free PMC article.
-
Unleashing Intrinsic Growth Pathways in Regenerating Peripheral Neurons.Int J Mol Sci. 2022 Nov 5;23(21):13566. doi: 10.3390/ijms232113566. Int J Mol Sci. 2022. PMID: 36362354 Free PMC article. Review.
-
Serum response factor promotes axon regeneration following spinal cord transection injury.Neural Regen Res. 2023 Sep;18(9):1956-1960. doi: 10.4103/1673-5374.367974. Neural Regen Res. 2023. PMID: 36926719 Free PMC article.
-
The Dynamics of Nerve Degeneration and Regeneration in a Healthy Milieu and in Diabetes.Int J Mol Sci. 2023 Oct 16;24(20):15241. doi: 10.3390/ijms242015241. Int J Mol Sci. 2023. PMID: 37894921 Free PMC article. Review.
References
-
- Neumann, S. and Woolf, C.J. (1999) Regeneration of dorsal column fibers into and beyond the lesion site following adult spinal cord injury. Neuron, 23, 83–91. - PubMed
-
- Fernandes, K.J., Fan, D.P., Tsui, B.J., Cassar, S.L. and Tetzlaff, W. (1999) Influence of the axotomy to cell body distance in rat rubrospinal and spinal motoneurons: differential regulation of GAP-43, tubulins, and neurofilament-M. J. Comp. Neurol., 414, 495–510. - PubMed
Publication types
MeSH terms
Substances
LinkOut - more resources
Full Text Sources
Molecular Biology Databases
Miscellaneous