Unravelling the mechanisms of Type 1A topoisomerases using single-molecule approaches
- PMID: 33963870
- PMCID: PMC8191776
- DOI: 10.1093/nar/gkab239
Unravelling the mechanisms of Type 1A topoisomerases using single-molecule approaches
Abstract
Topoisomerases are essential enzymes that regulate DNA topology. Type 1A family topoisomerases are found in nearly all living organisms and are unique in that they require single-stranded (ss)DNA for activity. These enzymes are vital for maintaining supercoiling homeostasis and resolving DNA entanglements generated during DNA replication and repair. While the catalytic cycle of Type 1A topoisomerases has been long-known to involve an enzyme-bridged ssDNA gate that allows strand passage, a deeper mechanistic understanding of these enzymes has only recently begun to emerge. This knowledge has been greatly enhanced through the combination of biochemical studies and increasingly sophisticated single-molecule assays based on magnetic tweezers, optical tweezers, atomic force microscopy and Förster resonance energy transfer. In this review, we discuss how single-molecule assays have advanced our understanding of the gate opening dynamics and strand-passage mechanisms of Type 1A topoisomerases, as well as the interplay of Type 1A topoisomerases with partner proteins, such as RecQ-family helicases. We also highlight how these assays have shed new light on the likely functional roles of Type 1A topoisomerases in vivo and discuss recent developments in single-molecule technologies that could be applied to further enhance our understanding of these essential enzymes.
© The Author(s) 2021. Published by Oxford University Press on behalf of Nucleic Acids Research.
Figures
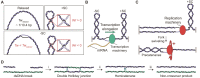
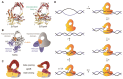
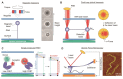
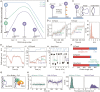
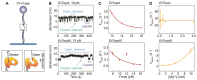
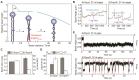
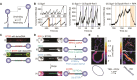

Similar articles
-
Duplex DNA and BLM regulate gate opening by the human TopoIIIα-RMI1-RMI2 complex.Nat Commun. 2022 Jan 31;13(1):584. doi: 10.1038/s41467-022-28082-5. Nat Commun. 2022. PMID: 35102151 Free PMC article.
-
RecQ helicase stimulates both DNA catenation and changes in DNA topology by topoisomerase III.J Biol Chem. 2003 Oct 24;278(43):42668-78. doi: 10.1074/jbc.M302994200. Epub 2003 Aug 8. J Biol Chem. 2003. PMID: 12909639
-
Roles of type 1A topoisomerases in genome maintenance in Escherichia coli.PLoS Genet. 2014 Aug 7;10(8):e1004543. doi: 10.1371/journal.pgen.1004543. eCollection 2014 Aug. PLoS Genet. 2014. PMID: 25102178 Free PMC article.
-
What's on the Other Side of the Gate: A Structural Perspective on DNA Gate Opening of Type IA and IIA DNA Topoisomerases.Int J Mol Sci. 2023 Feb 16;24(4):3986. doi: 10.3390/ijms24043986. Int J Mol Sci. 2023. PMID: 36835394 Free PMC article. Review.
-
Helicase-appended topoisomerases: new insight into the mechanism of directional strand transfer.J Biol Chem. 2009 Nov 6;284(45):30737-41. doi: 10.1074/jbc.R109.051268. Epub 2009 Sep 2. J Biol Chem. 2009. PMID: 19726668 Free PMC article. Review.
Cited by
-
A Novel Copper(II) Indenoisoquinoline Complex Inhibits Topoisomerase I, Induces G2 Phase Arrest, and Autophagy in Three Adenocarcinomas.Front Oncol. 2022 Feb 24;12:837373. doi: 10.3389/fonc.2022.837373. eCollection 2022. Front Oncol. 2022. PMID: 35280788 Free PMC article.
-
The interaction between transport-segment DNA and topoisomerase IA-crystal structure of MtbTOP1 in complex with both G- and T-segments.Nucleic Acids Res. 2023 Jan 11;51(1):349-364. doi: 10.1093/nar/gkac1205. Nucleic Acids Res. 2023. PMID: 36583363 Free PMC article.
-
Editorial: Single-molecule studies of DNA-protein interactions collection 2021.Nucleic Acids Res. 2021 Jun 21;49(11):6005-6006. doi: 10.1093/nar/gkab497. Nucleic Acids Res. 2021. PMID: 34153108 Free PMC article. No abstract available.
-
Variation of Structure and Cellular Functions of Type IA Topoisomerases across the Tree of Life.Cells. 2024 Mar 21;13(6):553. doi: 10.3390/cells13060553. Cells. 2024. PMID: 38534397 Free PMC article. Review.
-
The Implication of Topoisomerase II Inhibitors in Synthetic Lethality for Cancer Therapy.Pharmaceuticals (Basel). 2023 Jan 9;16(1):94. doi: 10.3390/ph16010094. Pharmaceuticals (Basel). 2023. PMID: 36678591 Free PMC article. Review.
References
-
- Bates A.D., Maxwell A.. DNA topology. 2005; Oxford University Press.
-
- Strick T.R., Allemand J.F., Bensimon D., Bensimon A., Croquette V.. The elasticity of a single supercoiled DNA molecule. Science. 1996; 271:1835–1837. - PubMed
Publication types
MeSH terms
Substances
LinkOut - more resources
Full Text Sources
Other Literature Sources
Research Materials