Reentrant liquid condensate phase of proteins is stabilized by hydrophobic and non-ionic interactions
- PMID: 33597515
- PMCID: PMC7889641
- DOI: 10.1038/s41467-021-21181-9
Reentrant liquid condensate phase of proteins is stabilized by hydrophobic and non-ionic interactions
Abstract
Liquid-liquid phase separation of proteins underpins the formation of membraneless compartments in living cells. Elucidating the molecular driving forces underlying protein phase transitions is therefore a key objective for understanding biological function and malfunction. Here we show that cellular proteins, which form condensates at low salt concentrations, including FUS, TDP-43, Brd4, Sox2, and Annexin A11, can reenter a phase-separated regime at high salt concentrations. By bringing together experiments and simulations, we demonstrate that this reentrant phase transition in the high-salt regime is driven by hydrophobic and non-ionic interactions, and is mechanistically distinct from the low-salt regime, where condensates are additionally stabilized by electrostatic forces. Our work thus sheds light on the cooperation of hydrophobic and non-ionic interactions as general driving forces in the condensation process, with important implications for aberrant function, druggability, and material properties of biomolecular condensates.
Conflict of interest statement
The authors declare no competing interests.
Figures
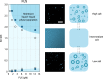
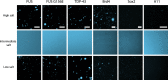
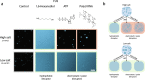
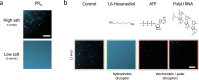
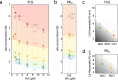
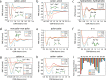
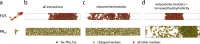
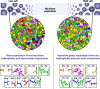
Similar articles
-
Single-Protein Collapse Determines Phase Equilibria of a Biological Condensate.J Phys Chem Lett. 2020 Jun 18;11(12):4923-4929. doi: 10.1021/acs.jpclett.0c01222. Epub 2020 Jun 9. J Phys Chem Lett. 2020. PMID: 32426986
-
Simulation of FUS Protein Condensates with an Adapted Coarse-Grained Model.J Chem Theory Comput. 2021 Jan 12;17(1):525-537. doi: 10.1021/acs.jctc.0c01064. Epub 2020 Dec 13. J Chem Theory Comput. 2021. PMID: 33307683 Free PMC article.
-
Atomistic insights into the reentrant phase-transitions in polyuracil and polylysine mixtures.J Chem Phys. 2024 Jul 7;161(1):015101. doi: 10.1063/5.0206190. J Chem Phys. 2024. PMID: 38949285
-
Modulating liquid-liquid phase separation of FUS: mechanisms and strategies.J Mater Chem B. 2022 Nov 3;10(42):8616-8628. doi: 10.1039/d2tb01688e. J Mater Chem B. 2022. PMID: 36268634 Review.
-
Biochemical Timekeeping Via Reentrant Phase Transitions.J Mol Biol. 2021 Jun 11;433(12):166794. doi: 10.1016/j.jmb.2020.166794. Epub 2020 Dec 31. J Mol Biol. 2021. PMID: 33387533 Free PMC article. Review.
Cited by
-
Learning the molecular grammar of protein condensates from sequence determinants and embeddings.Proc Natl Acad Sci U S A. 2021 Apr 13;118(15):e2019053118. doi: 10.1073/pnas.2019053118. Proc Natl Acad Sci U S A. 2021. PMID: 33827920 Free PMC article.
-
Disordered regions of human eIF4B orchestrate a dynamic self-association landscape.Nat Commun. 2024 Oct 10;15(1):8766. doi: 10.1038/s41467-024-53136-1. Nat Commun. 2024. PMID: 39384813 Free PMC article.
-
Improved coarse-grained model for studying sequence dependent phase separation of disordered proteins.Protein Sci. 2021 Jul;30(7):1371-1379. doi: 10.1002/pro.4094. Epub 2021 May 24. Protein Sci. 2021. PMID: 33934416 Free PMC article.
-
Ion binding with charge inversion combined with screening modulates DEAD box helicase phase transitions.Cell Rep. 2023 Nov 28;42(11):113375. doi: 10.1016/j.celrep.2023.113375. Epub 2023 Nov 18. Cell Rep. 2023. PMID: 37980572 Free PMC article.
-
Protein condensation diseases: therapeutic opportunities.Nat Commun. 2022 Sep 22;13(1):5550. doi: 10.1038/s41467-022-32940-7. Nat Commun. 2022. PMID: 36138006 Free PMC article. Review.
References
Publication types
MeSH terms
Substances
Associated data
Grants and funding
LinkOut - more resources
Full Text Sources
Other Literature Sources