The autophagic membrane tether ATG2A transfers lipids between membranes
- PMID: 31271352
- PMCID: PMC6625793
- DOI: 10.7554/eLife.45777
The autophagic membrane tether ATG2A transfers lipids between membranes
Abstract
An enigmatic step in de novo formation of the autophagosome membrane compartment is the expansion of the precursor membrane phagophore, which requires the acquisition of lipids to serve as building blocks. Autophagy-related 2 (ATG2), the rod-shaped protein that tethers phosphatidylinositol 3-phosphate (PI3P)-enriched phagophores to the endoplasmic reticulum (ER), is suggested to be essential for phagophore expansion, but the underlying mechanism remains unclear. Here, we demonstrate that human ATG2A is a lipid transfer protein. ATG2A can extract lipids from membrane vesicles and unload them to other vesicles. Lipid transfer by ATG2A is more efficient between tethered vesicles than between untethered vesicles. The PI3P effectors WIPI4 and WIPI1 associate ATG2A stably to PI3P-containing vesicles, thereby facilitating ATG2A-mediated tethering and lipid transfer between PI3P-containing vesicles and PI3P-free vesicles. Based on these results, we propose that ATG2-mediated transfer of lipids from the ER to the phagophore enables phagophore expansion.
Keywords: biochemistry; cell biology; chemical biology; human; isolation membrane; lipid transfer; membrane expansion; membrane tethering; organelle biogenesis; phagophore expansion.
© 2019, Maeda et al.
Conflict of interest statement
SM, CO, TO No competing interests declared
Figures
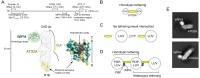
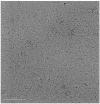
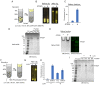
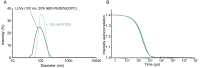
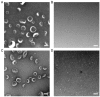
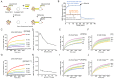
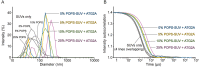
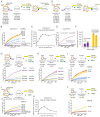
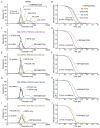
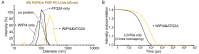
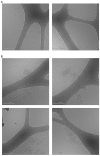
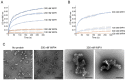
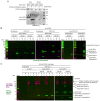
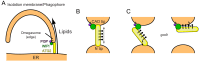
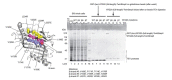
Comment in
-
ATG2A transfers lipids between membranes in vitro.Autophagy. 2019 Nov;15(11):2031-2032. doi: 10.1080/15548627.2019.1659622. Epub 2019 Aug 26. Autophagy. 2019. PMID: 31441376 Free PMC article.
Similar articles
-
Insights into autophagosome biogenesis from structural and biochemical analyses of the ATG2A-WIPI4 complex.Proc Natl Acad Sci U S A. 2018 Oct 16;115(42):E9792-E9801. doi: 10.1073/pnas.1811874115. Epub 2018 Sep 5. Proc Natl Acad Sci U S A. 2018. PMID: 30185561 Free PMC article.
-
The rod-shaped ATG2A-WIPI4 complex tethers membranes in vitro.Contact (Thousand Oaks). 2018 Jan-Dec;1:10.1177/2515256418819936. doi: 10.1177/2515256418819936. Epub 2018 Dec 21. Contact (Thousand Oaks). 2018. PMID: 30766969 Free PMC article.
-
TOM40 Targets Atg2 to Mitochondria-Associated ER Membranes for Phagophore Expansion.Cell Rep. 2019 Aug 13;28(7):1744-1757.e5. doi: 10.1016/j.celrep.2019.07.036. Cell Rep. 2019. PMID: 31412244 Free PMC article.
-
Atg2: A novel phospholipid transfer protein that mediates de novo autophagosome biogenesis.Protein Sci. 2019 Jun;28(6):1005-1012. doi: 10.1002/pro.3623. Epub 2019 Apr 29. Protein Sci. 2019. PMID: 30993752 Free PMC article. Review.
-
The Organization and Function of the Phagophore-ER Membrane Contact Sites.Contact (Thousand Oaks). 2023 Jul 12;6:25152564231183898. doi: 10.1177/25152564231183898. eCollection 2023 Jan-Dec. Contact (Thousand Oaks). 2023. PMID: 37465355 Free PMC article. Review.
Cited by
-
Autophagosome biogenesis comes out of the black box.Nat Cell Biol. 2021 May;23(5):450-456. doi: 10.1038/s41556-021-00669-y. Epub 2021 Apr 26. Nat Cell Biol. 2021. PMID: 33903736 Free PMC article. Review.
-
Endoplasmic Reticulum Membrane and Contact Site Dynamics in Autophagy Regulation and Stress Response.Front Cell Dev Biol. 2020 May 29;8:343. doi: 10.3389/fcell.2020.00343. eCollection 2020. Front Cell Dev Biol. 2020. PMID: 32548114 Free PMC article. Review.
-
Lipid osmosis, membrane tension, and other mechanochemical driving forces of lipid flow.bioRxiv [Preprint]. 2024 Apr 28:2024.01.08.574656. doi: 10.1101/2024.01.08.574656. bioRxiv. 2024. Update in: Curr Opin Cell Biol. 2024 Jun;88:102377. doi: 10.1016/j.ceb.2024.102377. PMID: 38260424 Free PMC article. Updated. Preprint.
-
Role of VPS13, a protein with similarity to ATG2, in physiology and disease.Curr Opin Genet Dev. 2020 Dec;65:61-68. doi: 10.1016/j.gde.2020.05.027. Epub 2020 Jun 18. Curr Opin Genet Dev. 2020. PMID: 32563856 Free PMC article. Review.
-
Atg8 family proteins, LIR/AIM motifs and other interaction modes.Autophagy Rep. 2023 Mar 19;2(1):27694127.2023.2188523. doi: 10.1080/27694127.2023.2188523. eCollection 2023 Dec 31. Autophagy Rep. 2023. PMID: 38214012 Free PMC article.
References
-
- Axe EL, Walker SA, Manifava M, Chandra P, Roderick HL, Habermann A, Griffiths G, Ktistakis NT. Autophagosome formation from membrane compartments enriched in phosphatidylinositol 3-phosphate and dynamically connected to the endoplasmic reticulum. The Journal of Cell Biology. 2008;182:685–701. doi: 10.1083/jcb.200803137. - DOI - PMC - PubMed
-
- Bakula D, Müller AJ, Zuleger T, Takacs Z, Franz-Wachtel M, Thost AK, Brigger D, Tschan MP, Frickey T, Robenek H, Macek B, Proikas-Cezanne T. WIPI3 and WIPI4 β-propellers are scaffolds for LKB1-AMPK-TSC signalling circuits in the control of autophagy. Nature Communications. 2017;8:15637. doi: 10.1038/ncomms15637. - DOI - PMC - PubMed
Publication types
MeSH terms
Substances
Grants and funding
LinkOut - more resources
Full Text Sources
Other Literature Sources
Molecular Biology Databases