Insights into autophagosome biogenesis from structural and biochemical analyses of the ATG2A-WIPI4 complex
- PMID: 30185561
- PMCID: PMC6196511
- DOI: 10.1073/pnas.1811874115
Insights into autophagosome biogenesis from structural and biochemical analyses of the ATG2A-WIPI4 complex
Abstract
Autophagy is an enigmatic cellular process in which double-membrane compartments, called "autophagosomes, form de novo adjacent to the endoplasmic reticulum (ER) and package cytoplasmic contents for delivery to lysosomes. Expansion of the precursor membrane phagophore requires autophagy-related 2 (ATG2), which localizes to the PI3P-enriched ER-phagophore junction. We combined single-particle electron microscopy, chemical cross-linking coupled with mass spectrometry, and biochemical analyses to characterize human ATG2A in complex with the PI3P effector WIPI4. ATG2A is a rod-shaped protein that can bridge neighboring vesicles through interactions at each of its tips. WIPI4 binds to one of the tips, enabling the ATG2A-WIPI4 complex to tether a PI3P-containing vesicle to another PI3P-free vesicle. These data suggest that the ATG2A-WIPI4 complex mediates ER-phagophore association and/or tethers vesicles to the ER-phagophore junction, establishing the required organization for phagophore expansion via the transfer of lipid membranes from the ER and/or the vesicles to the phagophore.
Keywords: ATG2; autophagy; chemical cross-linking coupled with mass spectrometry; membrane tethering; single-particle analysis.
Conflict of interest statement
The authors declare no conflict of interest.
Figures
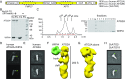
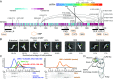
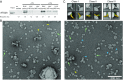
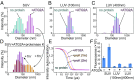
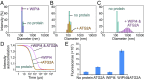
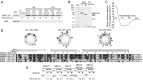
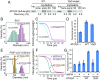
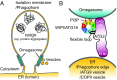
Comment in
-
Membrane tethering by the autophagy ATG2A-WIPI4 complex.Proc Natl Acad Sci U S A. 2018 Oct 16;115(42):10540-10541. doi: 10.1073/pnas.1814759115. Epub 2018 Oct 1. Proc Natl Acad Sci U S A. 2018. PMID: 30275332 Free PMC article. No abstract available.
Similar articles
-
The autophagic membrane tether ATG2A transfers lipids between membranes.Elife. 2019 Jul 4;8:e45777. doi: 10.7554/eLife.45777. Elife. 2019. PMID: 31271352 Free PMC article.
-
The rod-shaped ATG2A-WIPI4 complex tethers membranes in vitro.Contact (Thousand Oaks). 2018 Jan-Dec;1:10.1177/2515256418819936. doi: 10.1177/2515256418819936. Epub 2018 Dec 21. Contact (Thousand Oaks). 2018. PMID: 30766969 Free PMC article.
-
The Atg2-Atg18 complex tethers pre-autophagosomal membranes to the endoplasmic reticulum for autophagosome formation.Proc Natl Acad Sci U S A. 2018 Oct 9;115(41):10363-10368. doi: 10.1073/pnas.1806727115. Epub 2018 Sep 25. Proc Natl Acad Sci U S A. 2018. PMID: 30254161 Free PMC article.
-
Omegasomes control formation, expansion, and closure of autophagosomes.Bioessays. 2024 Jun;46(6):e2400038. doi: 10.1002/bies.202400038. Epub 2024 May 9. Bioessays. 2024. PMID: 38724256 Review.
-
Atg2: A novel phospholipid transfer protein that mediates de novo autophagosome biogenesis.Protein Sci. 2019 Jun;28(6):1005-1012. doi: 10.1002/pro.3623. Epub 2019 Apr 29. Protein Sci. 2019. PMID: 30993752 Free PMC article. Review.
Cited by
-
Vps13 is required for the packaging of the ER into autophagosomes during ER-phagy.Proc Natl Acad Sci U S A. 2020 Aug 4;117(31):18530-18539. doi: 10.1073/pnas.2008923117. Epub 2020 Jul 20. Proc Natl Acad Sci U S A. 2020. PMID: 32690699 Free PMC article.
-
MAMs and Mitochondrial Quality Control: Overview and Their Role in Alzheimer's Disease.Neurochem Res. 2024 Oct;49(10):2682-2698. doi: 10.1007/s11064-024-04205-w. Epub 2024 Jul 13. Neurochem Res. 2024. PMID: 39002091 Review.
-
Natural-Product-Mediated Autophagy in the Treatment of Various Liver Diseases.Int J Mol Sci. 2022 Dec 1;23(23):15109. doi: 10.3390/ijms232315109. Int J Mol Sci. 2022. PMID: 36499429 Free PMC article. Review.
-
CROP: a retromer-PROPPIN complex mediating membrane fission in the endo-lysosomal system.EMBO J. 2022 May 16;41(10):e109646. doi: 10.15252/embj.2021109646. Epub 2022 Apr 25. EMBO J. 2022. PMID: 35466426 Free PMC article.
-
Lipid Transport from Endoplasmic Reticulum to Autophagic Membranes.Cold Spring Harb Perspect Biol. 2022 Nov 1;14(11):a041254. doi: 10.1101/cshperspect.a041254. Cold Spring Harb Perspect Biol. 2022. PMID: 35940912 Free PMC article. Review.
References
-
- Mizushima N, Yoshimori T, Ohsumi Y. The role of Atg proteins in autophagosome formation. Annu Rev Cell Dev Biol. 2011;27:107–132. - PubMed
-
- Lamb CA, Yoshimori T, Tooze SA. The autophagosome: Origins unknown, biogenesis complex. Nat Rev Mol Cell Biol. 2013;14:759–774. - PubMed
-
- Mizushima N. A brief history of autophagy from cell biology to physiology and disease. Nat Cell Biol. 2018;20:521–527. - PubMed
Publication types
MeSH terms
Substances
Grants and funding
LinkOut - more resources
Full Text Sources
Other Literature Sources