Neural Circuit-Specialized Astrocytes: Transcriptomic, Proteomic, Morphological, and Functional Evidence
- PMID: 28712653
- PMCID: PMC5811312
- DOI: 10.1016/j.neuron.2017.06.029
Neural Circuit-Specialized Astrocytes: Transcriptomic, Proteomic, Morphological, and Functional Evidence
Abstract
Astrocytes are ubiquitous in the brain and are widely held to be largely identical. However, this view has not been fully tested, and the possibility that astrocytes are neural circuit specialized remains largely unexplored. Here, we used multiple integrated approaches, including RNA sequencing (RNA-seq), mass spectrometry, electrophysiology, immunohistochemistry, serial block-face-scanning electron microscopy, morphological reconstructions, pharmacogenetics, and diffusible dye, calcium, and glutamate imaging, to directly compare adult striatal and hippocampal astrocytes under identical conditions. We found significant differences in electrophysiological properties, Ca2+ signaling, morphology, and astrocyte-synapse proximity between striatal and hippocampal astrocytes. Unbiased evaluation of actively translated RNA and proteomic data confirmed significant astrocyte diversity between hippocampal and striatal circuits. We thus report core astrocyte properties, reveal evidence for specialized astrocytes within neural circuits, and provide new, integrated database resources and approaches to explore astrocyte diversity and function throughout the adult brain. VIDEO ABSTRACT.
Keywords: Aldh1l1; Cre/ERT2; GCaMP; RNA-seq; astrocyte; calcium; diversity; hippocampus; proteomics; striatum.
Copyright © 2017 Elsevier Inc. All rights reserved.
Figures
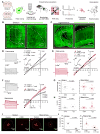
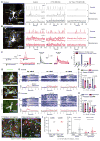
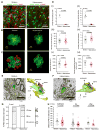
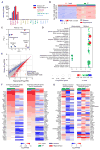
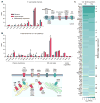
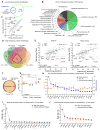
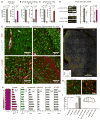
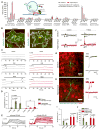
Comment in
-
Neurobiology: Diversity reaches the stars.Nature. 2017 Aug 23;548(7668):396-397. doi: 10.1038/548396a. Nature. 2017. PMID: 28836597 No abstract available.
-
Astrocytes: Tailored to Support the Demand of Neural Circuits?Cell Syst. 2017 Sep 27;5(3):165-167. doi: 10.1016/j.cels.2017.09.008. Cell Syst. 2017. PMID: 28957651
Similar articles
-
Dysfunctional Calcium and Glutamate Signaling in Striatal Astrocytes from Huntington's Disease Model Mice.J Neurosci. 2016 Mar 23;36(12):3453-70. doi: 10.1523/JNEUROSCI.3693-15.2016. J Neurosci. 2016. PMID: 27013675 Free PMC article.
-
New Transgenic Mouse Lines for Selectively Targeting Astrocytes and Studying Calcium Signals in Astrocyte Processes In Situ and In Vivo.Neuron. 2016 Dec 21;92(6):1181-1195. doi: 10.1016/j.neuron.2016.11.030. Epub 2016 Dec 8. Neuron. 2016. PMID: 27939582 Free PMC article.
-
Local and CNS-Wide Astrocyte Intracellular Calcium Signaling Attenuation In Vivo with CalExflox Mice.J Neurosci. 2021 May 26;41(21):4556-4574. doi: 10.1523/JNEUROSCI.0085-21.2021. Epub 2021 Apr 26. J Neurosci. 2021. PMID: 33903221 Free PMC article.
-
Local energy on demand: Are 'spontaneous' astrocytic Ca2+-microdomains the regulatory unit for astrocyte-neuron metabolic cooperation?Brain Res Bull. 2018 Jan;136:54-64. doi: 10.1016/j.brainresbull.2017.04.011. Epub 2017 Apr 24. Brain Res Bull. 2018. PMID: 28450076 Review.
-
Gliotransmission and the tripartite synapse.Adv Exp Med Biol. 2012;970:307-31. doi: 10.1007/978-3-7091-0932-8_14. Adv Exp Med Biol. 2012. PMID: 22351062 Review.
Cited by
-
Capacity of astrocytes to promote axon growth in the injured mammalian central nervous system.Front Neurosci. 2022 Sep 20;16:955598. doi: 10.3389/fnins.2022.955598. eCollection 2022. Front Neurosci. 2022. PMID: 36203815 Free PMC article. Review.
-
Insight into the role of phosphatidylserine in complement-mediated synapse loss in Alzheimer's disease.Fac Rev. 2021 Feb 24;10:19. doi: 10.12703/r/10-19. eCollection 2021. Fac Rev. 2021. PMID: 33718936 Free PMC article. Review.
-
Neuroimmunology of depression.Adv Pharmacol. 2021;91:259-292. doi: 10.1016/bs.apha.2021.03.004. Epub 2021 Apr 26. Adv Pharmacol. 2021. PMID: 34099111 Free PMC article.
-
Mapping Astrocyte Transcriptional Signatures in Response to Neuroactive Compounds.Int J Mol Sci. 2021 Apr 12;22(8):3975. doi: 10.3390/ijms22083975. Int J Mol Sci. 2021. PMID: 33921461 Free PMC article.
-
Neuronal subclass-selective proteomic analysis in Caenorhabditis elegans.Sci Rep. 2020 Aug 13;10(1):13840. doi: 10.1038/s41598-020-70692-w. Sci Rep. 2020. PMID: 32792517 Free PMC article.
References
-
- Bazargani N, Attwell D. Astrocyte calcium signalling: the third wave. Nat Neurosci. 2015 accepted. - PubMed
MeSH terms
Substances
Grants and funding
LinkOut - more resources
Full Text Sources
Other Literature Sources
Molecular Biology Databases
Research Materials
Miscellaneous