Brain metabolism in health, aging, and neurodegeneration
- PMID: 28438892
- PMCID: PMC5452017
- DOI: 10.15252/embj.201695810
Brain metabolism in health, aging, and neurodegeneration
Abstract
Brain cells normally respond adaptively to bioenergetic challenges resulting from ongoing activity in neuronal circuits, and from environmental energetic stressors such as food deprivation and physical exertion. At the cellular level, such adaptive responses include the "strengthening" of existing synapses, the formation of new synapses, and the production of new neurons from stem cells. At the molecular level, bioenergetic challenges result in the activation of transcription factors that induce the expression of proteins that bolster the resistance of neurons to the kinds of metabolic, oxidative, excitotoxic, and proteotoxic stresses involved in the pathogenesis of brain disorders including stroke, and Alzheimer's and Parkinson's diseases. Emerging findings suggest that lifestyles that include intermittent bioenergetic challenges, most notably exercise and dietary energy restriction, can increase the likelihood that the brain will function optimally and in the absence of disease throughout life. Here, we provide an overview of cellular and molecular mechanisms that regulate brain energy metabolism, how such mechanisms are altered during aging and in neurodegenerative disorders, and the potential applications to brain health and disease of interventions that engage pathways involved in neuronal adaptations to metabolic stress.
Keywords: aging; brain energetics; ketone bodies; metabolism.
Published 2017. This article is a U.S. Government work and is in the public domain in the USA.
Figures
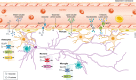
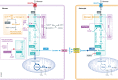
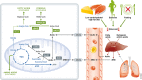
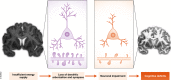
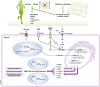
Similar articles
-
Adaptive responses of neuronal mitochondria to bioenergetic challenges: Roles in neuroplasticity and disease resistance.Free Radic Biol Med. 2017 Jan;102:203-216. doi: 10.1016/j.freeradbiomed.2016.11.045. Epub 2016 Nov 29. Free Radic Biol Med. 2017. PMID: 27908782 Free PMC article. Review.
-
Hallmarks of Brain Aging: Adaptive and Pathological Modification by Metabolic States.Cell Metab. 2018 Jun 5;27(6):1176-1199. doi: 10.1016/j.cmet.2018.05.011. Cell Metab. 2018. PMID: 29874566 Free PMC article. Review.
-
Modification of brain aging and neurodegenerative disorders by genes, diet, and behavior.Physiol Rev. 2002 Jul;82(3):637-72. doi: 10.1152/physrev.00004.2002. Physiol Rev. 2002. PMID: 12087131 Review.
-
A unifying hypothesis of Alzheimer's disease. IV. Causation and sequence of events.Rev Neurosci. 2000;11 Spec No:213-328. doi: 10.1515/revneuro.2000.11.s1.213. Rev Neurosci. 2000. PMID: 11065271 Review.
-
Energy intake and exercise as determinants of brain health and vulnerability to injury and disease.Cell Metab. 2012 Dec 5;16(6):706-22. doi: 10.1016/j.cmet.2012.08.012. Epub 2012 Nov 15. Cell Metab. 2012. PMID: 23168220 Free PMC article. Review.
Cited by
-
Glial Glutamine Homeostasis in Health and Disease.Neurochem Res. 2023 Apr;48(4):1100-1128. doi: 10.1007/s11064-022-03771-1. Epub 2022 Nov 2. Neurochem Res. 2023. PMID: 36322369 Review.
-
Glutamate-glutamine homeostasis is perturbed in neurons and astrocytes derived from patient iPSC models of frontotemporal dementia.Mol Brain. 2020 Sep 14;13(1):125. doi: 10.1186/s13041-020-00658-6. Mol Brain. 2020. PMID: 32928252 Free PMC article.
-
Lessons from the physiological role of guanosine in neurodegeneration and cancer: Toward a multimodal mechanism of action?Purinergic Signal. 2024 Jul 15. doi: 10.1007/s11302-024-10033-y. Online ahead of print. Purinergic Signal. 2024. PMID: 39004650 Review.
-
The contribution of astrocytes to obesity-associated metabolic disturbances.J Biomed Res. 2022 Aug 28;36(5):299-311. doi: 10.7555/JBR.36.20200020. J Biomed Res. 2022. PMID: 36131679 Free PMC article.
-
Neural ageing and synaptic plasticity: prioritizing brain health in healthy longevity.Front Aging Neurosci. 2024 Aug 5;16:1428244. doi: 10.3389/fnagi.2024.1428244. eCollection 2024. Front Aging Neurosci. 2024. PMID: 39161341 Free PMC article. Review.
References
-
- Abbott NJ, Patabendige AAK, Dolma DEM, Yusof SR, Begley DJ (2010) Structure and function of the blood‐brain barrier. Neurobiol Dis 37: 13–25 - PubMed
-
- Alle H, Roth A, Geiger JR (2009) Energy‐efficient action potentials in hippocampal mossy fibers. Science 325: 1405–1408 - PubMed
-
- Annunziata P, Volpi N (1985) High levels of C3c in the cerebrospinal fluid from amyotrophic lateral sclerosis patients. Acta Neurol Scand 72: 61–64 - PubMed
-
- Antonini A, Leenders KL, Spiegel R, Meier D, Vontobel P, Weigell‐Weber M, Sanchez‐Pernaute R, de Yébenez JG, Boesiger P, Weindl A, Maguire RP (1996) Striatal glucose metabolism and dopamine D2 receptor binding in asymptomatic gene carriers and patients with Huntington's disease. Brain 119: 2085–2095 - PubMed
-
- Attwell D, Laughlin SB (2001) An energy budget for signaling in the grey matter of the brain. J Cereb Blood Flow Metab 21: 1133–1145 - PubMed
Publication types
MeSH terms
LinkOut - more resources
Full Text Sources
Other Literature Sources
Medical