A Bioengineered Three-Dimensional Cell Culture Platform Integrated with Microfluidics To Address Antimicrobial Resistance in Tuberculosis
- PMID: 28174307
- PMCID: PMC5296599
- DOI: 10.1128/mBio.02073-16
A Bioengineered Three-Dimensional Cell Culture Platform Integrated with Microfluidics To Address Antimicrobial Resistance in Tuberculosis
Abstract
Antimicrobial resistance presents one of the most significant threats to human health, with the emergence of totally drug-resistant organisms. We have combined bioengineering, genetically modified bacteria, longitudinal readouts, and fluidics to develop a transformative platform to address the drug development bottleneck, utilizing Mycobacterium tuberculosis as the model organism. We generated microspheres incorporating virulent reporter bacilli, primary human cells, and an extracellular matrix by using bioelectrospray methodology. Granulomas form within the three-dimensional matrix, and mycobacterial stress genes are upregulated. Pyrazinamide, a vital first-line antibiotic for treating human tuberculosis, kills M. tuberculosis in a three-dimensional culture but not in a standard two-dimensional culture or Middlebrook 7H9 broth, demonstrating that antibiotic sensitivity within microspheres reflects conditions in patients. We then performed pharmacokinetic modeling by combining the microsphere system with a microfluidic plate and demonstrated that we can model the effect of dynamic antibiotic concentrations on mycobacterial killing. The microsphere system is highly tractable, permitting variation of cell content, the extracellular matrix, sphere size, the infectious dose, and the surrounding medium with the potential to address a wide array of human infections and the threat of antimicrobial resistance.
Importance: Antimicrobial resistance is a major global threat, and an emerging concept is that infection should be studied in the context of host immune cells. Tuberculosis is a chronic infection that kills over a million people every year and is becoming progressively more resistant to antibiotics. Recent major studies of shorter treatment or new vaccination approaches have not been successful, demonstrating that transformative technologies are required to control tuberculosis. We have developed an entirely new system to study the infection of host cells in a three-dimensional matrix by using bioengineering. We showed that antibiotics that work in patients are effective in this microsphere system but not in standard infection systems. We then combined microspheres with microfluidics to model drug concentration changes in patients and demonstrate the effect of increasing antibiotic concentrations on bacterial survival. This system can be widely applied to address the threat of antimicrobial resistance and develop new treatments.
Copyright © 2017 Bielecka et al.
Figures
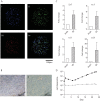
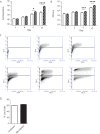
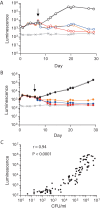
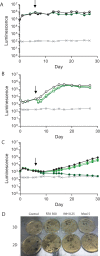
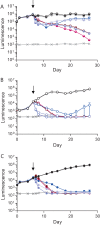
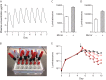
Similar articles
-
Dissection of the host-pathogen interaction in human tuberculosis using a bioengineered 3-dimensional model.Elife. 2017 Jan 7;6:e21283. doi: 10.7554/eLife.21283. Elife. 2017. PMID: 28063256 Free PMC article.
-
[Development of antituberculous drugs: current status and future prospects].Kekkaku. 2006 Dec;81(12):753-74. Kekkaku. 2006. PMID: 17240921 Review. Japanese.
-
Bioengineered 3D Models for Studying Human Cell-Tuberculosis Interactions.Trends Microbiol. 2017 Apr;25(4):245-246. doi: 10.1016/j.tim.2017.02.009. Epub 2017 Mar 8. Trends Microbiol. 2017. PMID: 28284875
-
Combating Antimicrobial Resistance via Single-Cell Diagnostic Technologies Powered by Droplet Microfluidics.Acc Chem Res. 2022 Jan 18;55(2):123-133. doi: 10.1021/acs.accounts.1c00462. Epub 2021 Dec 13. Acc Chem Res. 2022. PMID: 34898173 Free PMC article.
-
Tuberculosis.In: Holmes KK, Bertozzi S, Bloom BR, Jha P, editors. Major Infectious Diseases. 3rd edition. Washington (DC): The International Bank for Reconstruction and Development / The World Bank; 2017 Nov 3. Chapter 11. In: Holmes KK, Bertozzi S, Bloom BR, Jha P, editors. Major Infectious Diseases. 3rd edition. Washington (DC): The International Bank for Reconstruction and Development / The World Bank; 2017 Nov 3. Chapter 11. PMID: 30212088 Free Books & Documents. Review.
Cited by
-
Developments in the Diagnostic Techniques of Infectious Diseases: Rural and Urban Prospective.Adv Infect Dis. 2018 Sep;8(3):121-138. doi: 10.4236/aid.2018.83012. Epub 2018 Aug 23. Adv Infect Dis. 2018. PMID: 30197838 Free PMC article.
-
In Vitro Granuloma Models of Tuberculosis: Potential and Challenges.J Infect Dis. 2019 May 24;219(12):1858-1866. doi: 10.1093/infdis/jiz020. J Infect Dis. 2019. PMID: 30929010 Free PMC article. Review.
-
Integrated transcriptomic analysis of human tuberculosis granulomas and a biomimetic model identifies therapeutic targets.J Clin Invest. 2021 Aug 2;131(15):e148136. doi: 10.1172/JCI148136. J Clin Invest. 2021. PMID: 34128839 Free PMC article.
-
Drug permeation and metabolism in Mycobacterium tuberculosis: Prioritising local exposure as essential criterion in new TB drug development.IUBMB Life. 2018 Sep;70(9):926-937. doi: 10.1002/iub.1866. Epub 2018 Jun 22. IUBMB Life. 2018. PMID: 29934964 Free PMC article. Review.
-
Bioelectrospray Methodology for Dissection of the Host-pathogen Interaction in Human Tuberculosis.Bio Protoc. 2017 Jul 20;7(14):e2418. doi: 10.21769/BioProtoc.2418. Bio Protoc. 2017. PMID: 28904991 Free PMC article.
References
-
- WHO 2014. Antimicrobial resistance: global report on surveillance 2014. World Health Organization, Geneva, Switzerland: http://www.who.int/drugresistance/documents/surveillancereport/en/. Accessed 6 January 2016.
-
- Bush K, Courvalin P, Dantas G, Davies J, Eisenstein B, Huovinen P, Jacoby GA, Kishony R, Kreiswirth BN, Kutter E, Lerner SA, Levy S, Lewis K, Lomovskaya O, Miller JH, Mobashery S, Piddock LJ, Projan S, Thomas CM, Tomasz A, Tulkens PM, Walsh TR, Watson JD, Witkowski J, Witte W, Wright G, Yeh P, Zgurskaya HI. 2011. Tackling antibiotic resistance. Nat Rev Microbiol 9:894–896. doi:10.1038/nrmicro2693. - DOI - PMC - PubMed
Publication types
MeSH terms
Grants and funding
LinkOut - more resources
Full Text Sources
Other Literature Sources
Medical