Natural Killer Cells and Innate Interferon Gamma Participate in the Host Defense against Respiratory Vaccinia Virus Infection
- PMID: 26468539
- PMCID: PMC4702563
- DOI: 10.1128/JVI.01894-15
Natural Killer Cells and Innate Interferon Gamma Participate in the Host Defense against Respiratory Vaccinia Virus Infection
Abstract
In establishing a respiratory infection, vaccinia virus (VACV) initially replicates in airway epithelial cells before spreading to secondary sites of infection, mainly the draining lymph nodes, spleen, gastrointestinal tract, and reproductive organs. We recently reported that interferon gamma (IFN-γ) produced by CD8 T cells ultimately controls this disseminated infection, but the relative contribution of IFN-γ early in infection is unknown. Investigating the role of innate immune cells, we found that the frequency of natural killer (NK) cells in the lung increased dramatically between days 1 and 4 postinfection with VACV. Lung NK cells displayed an activated cell surface phenotype and were the primary source of IFN-γ prior to the arrival of CD8 T cells. In the presence of an intact CD8 T cell compartment, depletion of NK cells resulted in increased lung viral load at the time of peak disease severity but had no effect on eventual viral clearance, disease symptoms, or survival. In sharp contrast, RAG(-/-) mice devoid of T cells failed to control VACV and succumbed to infection despite a marked increase in NK cells in the lung. Supporting an innate immune role for NK cell-derived IFN-γ, we found that NK cell-depleted or IFN-γ-depleted RAG(-/-) mice displayed increased lung VACV titers and dissemination to ovaries and a significantly shorter mean time to death compared to untreated NK cell-competent RAG(-/-) controls. Together, these findings demonstrate a role for IFN-γ in aspects of both the innate and adaptive immune response to VACV and highlight the importance of NK cells in T cell-independent control of VACV in the respiratory tract.
Importance: Herein, we provide the first systematic evaluation of natural killer (NK) cell function in the lung after infection with vaccinia virus, a member of the Poxviridae family. The respiratory tract is an important mucosal site for entry of many human pathogens, including poxviruses, but precisely how our immune system defends the lung against these invaders remains unclear. Natural killer cells are a type of cytotoxic lymphocyte and part of our innate immune system. In recent years, NK cells have received increasing levels of attention following the discovery that different tissues contain specific subsets of NK cells with distinctive phenotypes and function. They are abundant in the lung, but their role in defense against respiratory viruses is poorly understood. What this study demonstrates is that NK cells are recruited, activated, and contribute to protection of the lung during a severe respiratory infection with vaccinia virus.
Copyright © 2015, American Society for Microbiology. All Rights Reserved.
Figures
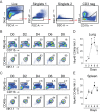
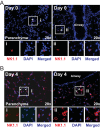
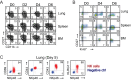
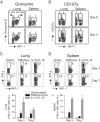
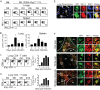
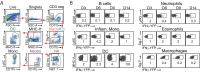
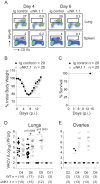
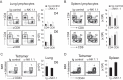
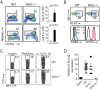
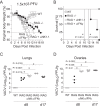
Similar articles
-
Insufficient Innate Immunity Contributes to the Susceptibility of the Castaneous Mouse to Orthopoxvirus Infection.J Virol. 2017 Sep 12;91(19):e01042-17. doi: 10.1128/JVI.01042-17. Print 2017 Oct 1. J Virol. 2017. PMID: 28747505 Free PMC article.
-
CD69 Deficiency Enhances the Host Response to Vaccinia Virus Infection through Altered NK Cell Homeostasis.J Virol. 2016 Jun 24;90(14):6464-6474. doi: 10.1128/JVI.00550-16. Print 2016 Jul 15. J Virol. 2016. PMID: 27147744 Free PMC article.
-
Selective reconstitution of IFN‑γ gene function in Ncr1+ NK cells is sufficient to control systemic vaccinia virus infection.PLoS Pathog. 2020 Feb 5;16(2):e1008279. doi: 10.1371/journal.ppat.1008279. eCollection 2020 Feb. PLoS Pathog. 2020. PMID: 32023327 Free PMC article.
-
Innate and adaptive immune responses that control lymph-borne viruses in the draining lymph node.Cell Mol Immunol. 2024 Sep;21(9):999-1007. doi: 10.1038/s41423-024-01188-0. Epub 2024 Jun 25. Cell Mol Immunol. 2024. PMID: 38918577 Free PMC article. Review.
-
Vaccinia Virus: Mechanisms Supporting Immune Evasion and Successful Long-Term Protective Immunity.Viruses. 2024 May 29;16(6):870. doi: 10.3390/v16060870. Viruses. 2024. PMID: 38932162 Free PMC article. Review.
Cited by
-
NK Cell IL-10 Production Requires IL-15 and IL-10 Driven STAT3 Activation.Front Immunol. 2019 Sep 4;10:2087. doi: 10.3389/fimmu.2019.02087. eCollection 2019. Front Immunol. 2019. PMID: 31552035 Free PMC article.
-
The host immune response in respiratory virus infection: balancing virus clearance and immunopathology.Semin Immunopathol. 2016 Jul;38(4):471-82. doi: 10.1007/s00281-016-0558-0. Epub 2016 Mar 10. Semin Immunopathol. 2016. PMID: 26965109 Free PMC article. Review.
-
Innate Immune Response to Monkeypox Virus Infection: Mechanisms and Immune Escape.J Innate Immun. 2024;16(1):413-424. doi: 10.1159/000540815. Epub 2024 Aug 13. J Innate Immun. 2024. PMID: 39137733 Free PMC article. Review.
-
NK cells contribute to reovirus-induced IFN responses and loss of tolerance to dietary antigen.JCI Insight. 2022 Aug 22;7(16):e159823. doi: 10.1172/jci.insight.159823. JCI Insight. 2022. PMID: 35993365 Free PMC article.
-
Batf3-Dependent Dendritic Cells Promote Optimal CD8 T Cell Responses against Respiratory Poxvirus Infection.J Virol. 2018 Jul 31;92(16):e00495-18. doi: 10.1128/JVI.00495-18. Print 2018 Aug 15. J Virol. 2018. PMID: 29875235 Free PMC article.
References
-
- de Souza Trindade G, Drumond BP, Guedes MI, Leite JA, Mota BE, Campos MA, da Fonseca FG, Nogueira ML, Lobato ZI, Bonjardim CA, Ferreira PC, Kroon EG. 2007. Zoonotic vaccinia virus infection in Brazil: clinical description and implications for health professionals. J Clin Microbiol 45:1370–1372. doi:10.1128/JCM.00920-06. - DOI - PMC - PubMed
Publication types
MeSH terms
Substances
Grants and funding
LinkOut - more resources
Full Text Sources
Molecular Biology Databases
Research Materials