A viral packaging motor varies its DNA rotation and step size to preserve subunit coordination as the capsid fills
- PMID: 24766813
- PMCID: PMC4003460
- DOI: 10.1016/j.cell.2014.02.034
A viral packaging motor varies its DNA rotation and step size to preserve subunit coordination as the capsid fills
Abstract
Multimeric, ring-shaped molecular motors rely on the coordinated action of their subunits to perform crucial biological functions. During these tasks, motors often change their operation in response to regulatory signals. Here, we investigate a viral packaging machine as it fills the capsid with DNA and encounters increasing internal pressure. We find that the motor rotates the DNA during packaging and that the rotation per base pair increases with filling. This change accompanies a reduction in the motor's step size. We propose that these adjustments preserve motor coordination by allowing one subunit to make periodic, specific, and regulatory contacts with the DNA. At high filling, we also observe the downregulation of the ATP-binding rate and the emergence of long-lived pauses, suggesting a throttling-down mechanism employed by the motor near the completion of packaging. This study illustrates how a biological motor adjusts its operation in response to changing conditions, while remaining highly coordinated.
Copyright © 2014 Elsevier Inc. All rights reserved.
Figures
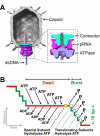
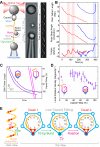
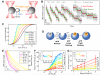
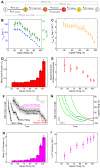
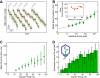
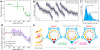
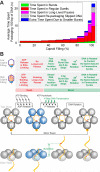
Similar articles
-
Substrate interactions and promiscuity in a viral DNA packaging motor.Nature. 2009 Oct 1;461(7264):669-73. doi: 10.1038/nature08443. Nature. 2009. PMID: 19794496 Free PMC article.
-
An RNA Domain Imparts Specificity and Selectivity to a Viral DNA Packaging Motor.J Virol. 2015 Dec;89(24):12457-66. doi: 10.1128/JVI.01895-15. Epub 2015 Sep 30. J Virol. 2015. PMID: 26423956 Free PMC article.
-
"Push through one-way valve" mechanism of viral DNA packaging.Adv Virus Res. 2012;83:415-65. doi: 10.1016/B978-0-12-394438-2.00009-8. Adv Virus Res. 2012. PMID: 22748815 Review.
-
Interaction of gp16 with pRNA and DNA for genome packaging by the motor of bacterial virus phi29.J Mol Biol. 2006 Feb 24;356(3):589-99. doi: 10.1016/j.jmb.2005.10.045. Epub 2005 Nov 9. J Mol Biol. 2006. PMID: 16376938
-
Bacterial virus phi29 DNA-packaging motor and its potential applications in gene therapy and nanotechnology.Methods Mol Biol. 2005;300:285-324. doi: 10.1385/1-59259-858-7:285. Methods Mol Biol. 2005. PMID: 15657489 Review.
Cited by
-
Using a system's equilibrium behavior to reduce its energy dissipation in nonequilibrium processes.Proc Natl Acad Sci U S A. 2019 Mar 26;116(13):5920-5924. doi: 10.1073/pnas.1817778116. Epub 2019 Mar 13. Proc Natl Acad Sci U S A. 2019. PMID: 30867295 Free PMC article.
-
Molecular switch-like regulation in motor proteins.Philos Trans R Soc Lond B Biol Sci. 2018 Jun 19;373(1749):20170181. doi: 10.1098/rstb.2017.0181. Philos Trans R Soc Lond B Biol Sci. 2018. PMID: 29735735 Free PMC article. Review.
-
Anterograde Viral Tracer Herpes Simplex Virus 1 Strain H129 Transports Primarily as Capsids in Cortical Neuron Axons.J Virol. 2020 Mar 31;94(8):e01957-19. doi: 10.1128/JVI.01957-19. Print 2020 Mar 31. J Virol. 2020. PMID: 31969440 Free PMC article.
-
Mechanochemical coupling and bi-phasic force-velocity dependence in the ultra-fast ring ATPase SpoIIIE.Elife. 2018 Mar 5;7:e32354. doi: 10.7554/eLife.32354. Elife. 2018. PMID: 29504934 Free PMC article.
-
Nonequilibrium dynamics and ultraslow relaxation of confined DNA during viral packaging.Proc Natl Acad Sci U S A. 2014 Jun 10;111(23):8345-50. doi: 10.1073/pnas.1405109111. Epub 2014 May 27. Proc Natl Acad Sci U S A. 2014. PMID: 24912187 Free PMC article.
References
-
- Berg HC. The rotary motor of bacterial flagella. Annu Rev Biochem. 2003;72:19–54. - PubMed
-
- Bryant Z, Stone MD, Gore J, Smith SB, Cozzarelli NR, Bustamante C. Structural transitions and elasticity from torque measurements on DNA. Nature. 2003;424:338–341. - PubMed
-
- Bustamante C, Marko JF, Siggia ED, Smith S. Entropic elasticity of lambda-phage DNA. Science. 1994;265:1599–1600. - PubMed
Publication types
MeSH terms
Substances
Grants and funding
LinkOut - more resources
Full Text Sources
Other Literature Sources