Microbial and animal rhodopsins: structures, functions, and molecular mechanisms
- PMID: 24364740
- PMCID: PMC3979449
- DOI: 10.1021/cr4003769
Microbial and animal rhodopsins: structures, functions, and molecular mechanisms
Figures
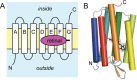
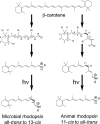
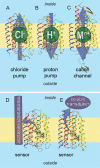
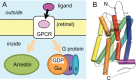
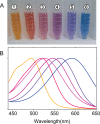
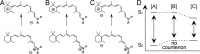
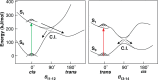
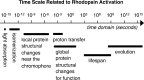
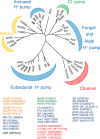
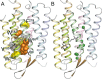
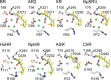
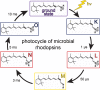
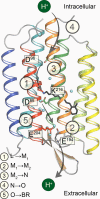
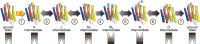
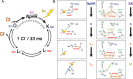
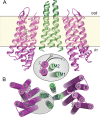
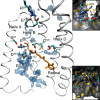
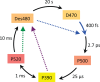
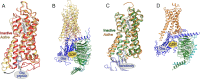
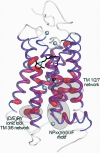
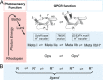
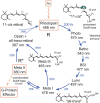
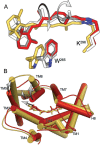
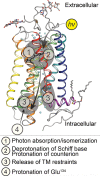
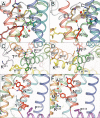
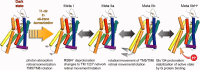
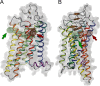
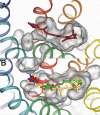
Similar articles
-
Light-driven ion-translocating rhodopsins in marine bacteria.Trends Microbiol. 2015 Feb;23(2):91-8. doi: 10.1016/j.tim.2014.10.009. Trends Microbiol. 2015. PMID: 25432080 Review.
-
Eubacterial rhodopsins - unique photosensors and diverse ion pumps.Biochim Biophys Acta. 2014 May;1837(5):553-61. doi: 10.1016/j.bbabio.2013.05.006. Epub 2013 Jun 6. Biochim Biophys Acta. 2014. PMID: 23748216 Review.
-
Crossing the borders: archaeal rhodopsins go bacterial.Trends Microbiol. 2003 Sep;11(9):405-7. doi: 10.1016/s0966-842x(03)00203-8. Trends Microbiol. 2003. PMID: 13678852 No abstract available.
-
Microbial Rhodopsins.Methods Mol Biol. 2022;2501:1-52. doi: 10.1007/978-1-0716-2329-9_1. Methods Mol Biol. 2022. PMID: 35857221
-
Ion-pumping microbial rhodopsin protein classification by machine learning approach.BMC Bioinformatics. 2023 Jan 27;24(1):29. doi: 10.1186/s12859-023-05138-x. BMC Bioinformatics. 2023. PMID: 36707759 Free PMC article.
Cited by
-
Optogenetic restoration of high sensitivity vision with bReaChES, a red-shifted channelrhodopsin.Sci Rep. 2022 Nov 11;12(1):19312. doi: 10.1038/s41598-022-23572-4. Sci Rep. 2022. PMID: 36369267 Free PMC article.
-
The Desensitized Channelrhodopsin-2 Photointermediate Contains 13 -cis, 15 -syn Retinal Schiff Base.Angew Chem Int Ed Engl. 2021 Jul 19;60(30):16442-16447. doi: 10.1002/anie.202015797. Epub 2021 Jun 17. Angew Chem Int Ed Engl. 2021. PMID: 33973334 Free PMC article.
-
Fluorescence of the Retinal Chromophore in Microbial and Animal Rhodopsins.Int J Mol Sci. 2023 Dec 8;24(24):17269. doi: 10.3390/ijms242417269. Int J Mol Sci. 2023. PMID: 38139098 Free PMC article. Review.
-
Characterization of an Unconventional Rhodopsin from the Freshwater Actinobacterium Rhodoluna lacicola.J Bacteriol. 2015 Aug;197(16):2704-12. doi: 10.1128/JB.00386-15. Epub 2015 Jun 8. J Bacteriol. 2015. PMID: 26055118 Free PMC article.
-
Early-stage dynamics of chloride ion-pumping rhodopsin revealed by a femtosecond X-ray laser.Proc Natl Acad Sci U S A. 2021 Mar 30;118(13):e2020486118. doi: 10.1073/pnas.2020486118. Proc Natl Acad Sci U S A. 2021. PMID: 33753488 Free PMC article.
References
Publication types
MeSH terms
Substances
Grants and funding
LinkOut - more resources
Full Text Sources
Other Literature Sources