Large-scale, high-density (up to 512 channels) recording of local circuits in behaving animals
- PMID: 24353300
- PMCID: PMC3949233
- DOI: 10.1152/jn.00785.2013
Large-scale, high-density (up to 512 channels) recording of local circuits in behaving animals
Abstract
Monitoring representative fractions of neurons from multiple brain circuits in behaving animals is necessary for understanding neuronal computation. Here, we describe a system that allows high-channel-count recordings from a small volume of neuronal tissue using a lightweight signal multiplexing headstage that permits free behavior of small rodents. The system integrates multishank, high-density recording silicon probes, ultraflexible interconnects, and a miniaturized microdrive. These improvements allowed for simultaneous recordings of local field potentials and unit activity from hundreds of sites without confining free movements of the animal. The advantages of large-scale recordings are illustrated by determining the electroanatomic boundaries of layers and regions in the hippocampus and neocortex and constructing a circuit diagram of functional connections among neurons in real anatomic space. These methods will allow the investigation of circuit operations and behavior-dependent interregional interactions for testing hypotheses of neural networks and brain function.
Keywords: behaving rats and mice; local field potential; monosynaptic connections; unit firing.
Figures
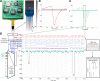
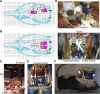
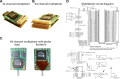
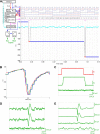
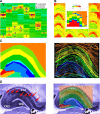
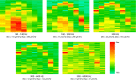
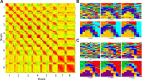
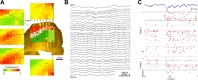
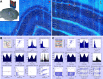
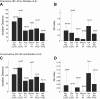
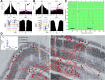
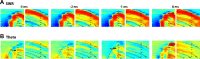
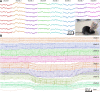
Similar articles
-
Integration of silicon-based neural probes and micro-drive arrays for chronic recording of large populations of neurons in behaving animals.J Neural Eng. 2016 Aug;13(4):046018. doi: 10.1088/1741-2560/13/4/046018. Epub 2016 Jun 28. J Neural Eng. 2016. PMID: 27351591
-
Design of a twin tetrode microdrive and headstage for hippocampal single unit recordings in behaving mice.J Neurosci Methods. 2003 Oct 30;129(2):129-34. doi: 10.1016/s0165-0270(03)00172-9. J Neurosci Methods. 2003. PMID: 14511816
-
Large-scale chronically implantable precision motorized microdrive array for freely behaving animals.J Neurophysiol. 2008 Oct;100(4):2430-40. doi: 10.1152/jn.90687.2008. Epub 2008 Jul 30. J Neurophysiol. 2008. PMID: 18667539 Free PMC article.
-
Tools for probing local circuits: high-density silicon probes combined with optogenetics.Neuron. 2015 Apr 8;86(1):92-105. doi: 10.1016/j.neuron.2015.01.028. Neuron. 2015. PMID: 25856489 Free PMC article. Review.
-
Imaging neuronal populations in behaving rodents: paradigms for studying neural circuits underlying behavior in the mammalian cortex.J Neurosci. 2013 Nov 6;33(45):17631-40. doi: 10.1523/JNEUROSCI.3255-13.2013. J Neurosci. 2013. PMID: 24198355 Free PMC article. Review.
Cited by
-
Internally organized mechanisms of the head direction sense.Nat Neurosci. 2015 Apr;18(4):569-75. doi: 10.1038/nn.3968. Epub 2015 Mar 2. Nat Neurosci. 2015. PMID: 25730672 Free PMC article.
-
Inferring thalamocortical monosynaptic connectivity in vivo.J Neurophysiol. 2021 Jun 1;125(6):2408-2431. doi: 10.1152/jn.00591.2020. Epub 2021 May 12. J Neurophysiol. 2021. PMID: 33978507 Free PMC article.
-
Grid cell remapping under three-dimensional object and social landmarks detected by implantable microelectrode arrays for the medial entorhinal cortex.Microsyst Nanoeng. 2022 Sep 16;8:104. doi: 10.1038/s41378-022-00436-5. eCollection 2022. Microsyst Nanoeng. 2022. PMID: 36124081 Free PMC article.
-
Recording extracellular neural activity in the behaving monkey using a semichronic and high-density electrode system.J Neurophysiol. 2016 Aug 1;116(2):563-74. doi: 10.1152/jn.00116.2016. Epub 2016 May 11. J Neurophysiol. 2016. PMID: 27169505 Free PMC article.
-
Flexible switch matrix addressable electrode arrays with organic electrochemical transistor and pn diode technology.Nat Commun. 2024 Jan 15;15(1):533. doi: 10.1038/s41467-023-44024-1. Nat Commun. 2024. PMID: 38225257 Free PMC article.
References
-
- Barthó P, Hirase H, Monconduit L, Zugaro M, Harris KD, Buzsáki G. Characterization of neocortical principal cells and interneurons by network interactions and extracellular features. J Neurophysiol 92: 600–608, 2004 - PubMed
Publication types
MeSH terms
Grants and funding
LinkOut - more resources
Full Text Sources
Other Literature Sources