Stem cell metabolism in tissue development and aging
- PMID: 23715547
- PMCID: PMC3666381
- DOI: 10.1242/dev.091777
Stem cell metabolism in tissue development and aging
Abstract
Recent advances in metabolomics and computational analysis have deepened our appreciation for the role of specific metabolic pathways in dictating cell fate. Once thought to be a mere consequence of the state of a cell, metabolism is now known to play a pivotal role in dictating whether a cell proliferates, differentiates or remains quiescent. Here, we review recent studies of metabolism in stem cells that have revealed a shift in the balance between glycolysis, mitochondrial oxidative phosphorylation and oxidative stress during the maturation of adult stem cells, and during the reprogramming of somatic cells to pluripotency. These insights promise to inform strategies for the directed differentiation of stem cells and to offer the potential for novel metabolic or pharmacological therapies to enhance regeneration and the treatment of degenerative disease.
Keywords: Blastocyst metabolism; Hematopoietic progenitors; Metabolomics; Pluripotent stem cells; Reprogramming.
Figures
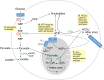
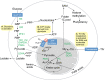
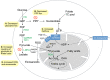
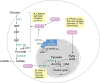
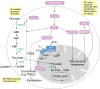
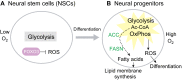
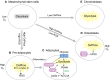
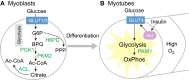
Similar articles
-
Connecting Mitochondria, Metabolism, and Stem Cell Fate.Stem Cells Dev. 2015 Sep 1;24(17):1957-71. doi: 10.1089/scd.2015.0117. Epub 2015 Jul 2. Stem Cells Dev. 2015. PMID: 26134242 Free PMC article. Review.
-
Combinatorial metabolism drives the naive to primed pluripotent chromatin landscape.Exp Cell Res. 2020 Apr 15;389(2):111913. doi: 10.1016/j.yexcr.2020.111913. Epub 2020 Feb 19. Exp Cell Res. 2020. PMID: 32084392 Review.
-
Metabolic Reprogramming of Stem Cell Epigenetics.Cell Stem Cell. 2015 Dec 3;17(6):651-662. doi: 10.1016/j.stem.2015.11.012. Cell Stem Cell. 2015. PMID: 26637942 Free PMC article. Review.
-
Somatic oxidative bioenergetics transitions into pluripotency-dependent glycolysis to facilitate nuclear reprogramming.Cell Metab. 2011 Aug 3;14(2):264-71. doi: 10.1016/j.cmet.2011.06.011. Cell Metab. 2011. PMID: 21803296 Free PMC article.
-
Mechanisms of the Metabolic Shift during Somatic Cell Reprogramming.Int J Mol Sci. 2019 May 7;20(9):2254. doi: 10.3390/ijms20092254. Int J Mol Sci. 2019. PMID: 31067778 Free PMC article. Review.
Cited by
-
An enduring role for quiescent stem cells.Dev Dyn. 2016 Jul;245(7):718-26. doi: 10.1002/dvdy.24416. Epub 2016 Jun 1. Dev Dyn. 2016. PMID: 27153394 Free PMC article. Review.
-
Metabolism and the Control of Cell Fate Decisions and Stem Cell Renewal.Annu Rev Cell Dev Biol. 2016 Oct 6;32:399-409. doi: 10.1146/annurev-cellbio-111315-125134. Epub 2016 Aug 1. Annu Rev Cell Dev Biol. 2016. PMID: 27482603 Free PMC article. Review.
-
Glycolysis regulates Hedgehog signalling via the plasma membrane potential.EMBO J. 2020 Nov 2;39(21):e101767. doi: 10.15252/embj.2019101767. Epub 2020 Oct 6. EMBO J. 2020. PMID: 33021744 Free PMC article.
-
Molecular mechanisms of cellular metabolic homeostasis in stem cells.Int J Oral Sci. 2023 Dec 1;15(1):52. doi: 10.1038/s41368-023-00262-z. Int J Oral Sci. 2023. PMID: 38040705 Free PMC article. Review.
-
Rapid, Dynamic Activation of Müller Glial Stem Cell Responses in Zebrafish.Invest Ophthalmol Vis Sci. 2016 Oct 1;57(13):5148-5160. doi: 10.1167/iovs.16-19973. Invest Ophthalmol Vis Sci. 2016. PMID: 27699411 Free PMC article.
References
-
- Ambros V., Horvitz H. R. (1984). Heterochronic mutants of the nematode Caenorhabditis elegans. Science 226, 409-416 - PubMed
-
- Arantes-Oliveira N., Apfeld J., Dillin A., Kenyon C. (2002). Regulation of life-span by germ-line stem cells in Caenorhabditis elegans. Science 295, 502-505 - PubMed
-
- Azuara V., Perry P., Sauer S., Spivakov M., Jørgensen H. F., John R. M., Gouti M., Casanova M., Warnes G., Merkenschlager M., et al. (2006). Chromatin signatures of pluripotent cell lines. Nat. Cell Biol. 8, 532-538 - PubMed
Publication types
MeSH terms
LinkOut - more resources
Full Text Sources
Other Literature Sources