Adenosinergic depression of glutamatergic transmission in the entorhinal cortex of juvenile rats via reduction of glutamate release probability and the number of releasable vesicles
- PMID: 23614032
- PMCID: PMC3628342
- DOI: 10.1371/journal.pone.0062185
Adenosinergic depression of glutamatergic transmission in the entorhinal cortex of juvenile rats via reduction of glutamate release probability and the number of releasable vesicles
Abstract
Adenosine is an inhibitory neuromodulator that exerts antiepileptic effects in the brain and the entorhinal cortex (EC) is an essential structure involved in temporal lobe epilepsy. Whereas microinjection of adenosine into the EC has been shown to exert powerful antiepileptic effects, the underlying cellular and molecular mechanisms in the EC have not been determined yet. We tested the hypothesis that adenosine-mediated modulation of synaptic transmission contributes to its antiepileptic effects in the EC. Our results demonstrate that adenosine reversibly inhibited glutamatergic transmission via activation of adenosine A1 receptors without effects on GABAergic transmission in layer III pyramidal neurons in the EC. Adenosine-induced depression of glutamatergic transmission was mediated by inhibiting presynaptic glutamate release probability and decreasing the number of readily releasable vesicles. Bath application of adenosine also reduced the frequency of the miniature EPSCs recorded in the presence of TTX suggesting that adenosine may interact with the exocytosis processes downstream of Ca(2+) influx. Both Gαi/o proteins and the protein kinase A pathway were required for adenosine-induced depression of glutamatergic transmission. We further showed that bath application of picrotoxin to the EC slices induced stable epileptiform activity and bath application of adenosine dose-dependently inhibited the epileptiform activity in this seizure model. Adenosine-mediated depression of epileptiform activity was mediated by activation of adenosine A1 receptors and required the functions of Gαi/o proteins and protein kinase A pathway. Our results suggest that the depression of glutamatergic transmission induced by adenosine contributes to its antiepileptic effects in the EC.
Conflict of interest statement
Figures
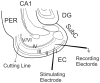
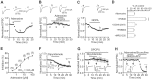
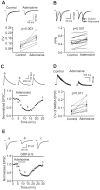
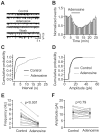
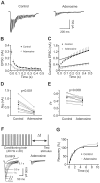
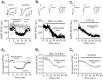
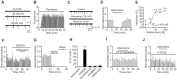
Similar articles
-
Adenosine modulates the excitability of layer II stellate neurons in entorhinal cortex through A1 receptors.Hippocampus. 2011 Mar;21(3):265-80. doi: 10.1002/hipo.20745. Hippocampus. 2011. PMID: 20054814
-
Activation of group II metabotropic glutamate receptors inhibits glutamatergic transmission in the rat entorhinal cortex via reduction of glutamate release probability.Cereb Cortex. 2012 Mar;22(3):584-94. doi: 10.1093/cercor/bhr131. Epub 2011 Jun 15. Cereb Cortex. 2012. PMID: 21677028 Free PMC article.
-
Enhanced actions of adenosine in medial entorhinal cortex layer II stellate neurons in temporal lobe epilepsy are mediated via A(1)-receptor activation.Epilepsia. 2012 Jan;53(1):168-76. doi: 10.1111/j.1528-1167.2011.03337.x. Epub 2011 Nov 29. Epilepsia. 2012. PMID: 22126400 Free PMC article.
-
Serotonin increases GABA release in rat entorhinal cortex by inhibiting interneuron TASK-3 K+ channels.Mol Cell Neurosci. 2008 Oct;39(2):273-84. doi: 10.1016/j.mcn.2008.07.005. Epub 2008 Jul 18. Mol Cell Neurosci. 2008. PMID: 18687403 Free PMC article.
-
Bombesin facilitates GABAergic transmission and depresses epileptiform activity in the entorhinal cortex.Hippocampus. 2014 Jan;24(1):21-31. doi: 10.1002/hipo.22191. Epub 2013 Sep 14. Hippocampus. 2014. PMID: 23966303
Cited by
-
The Signaling Pathways Involved in the Anticonvulsive Effects of the Adenosine A1 Receptor.Int J Mol Sci. 2020 Dec 30;22(1):320. doi: 10.3390/ijms22010320. Int J Mol Sci. 2020. PMID: 33396826 Free PMC article. Review.
-
Adenosine receptors and epilepsy: current evidence and future potential.Int Rev Neurobiol. 2014;119:233-55. doi: 10.1016/B978-0-12-801022-8.00011-8. Int Rev Neurobiol. 2014. PMID: 25175969 Free PMC article. Review.
-
Astrocytes control synaptic strength by two distinct v-SNARE-dependent release pathways.Nat Neurosci. 2017 Nov;20(11):1529-1539. doi: 10.1038/nn.4647. Epub 2017 Sep 25. Nat Neurosci. 2017. PMID: 28945220
-
Neurotensinergic augmentation of glutamate release at the perforant path-granule cell synapse in rat dentate gyrus: Roles of L-Type Ca²⁺ channels, calmodulin and myosin light-chain kinase.Neuropharmacology. 2015 Aug;95:252-60. doi: 10.1016/j.neuropharm.2015.03.028. Epub 2015 Apr 2. Neuropharmacology. 2015. PMID: 25842242 Free PMC article.
-
Adenosine Differentially Modulates Synaptic Transmission of Excitatory and Inhibitory Microcircuits in Layer 4 of Rat Barrel Cortex.Cereb Cortex. 2017 Sep 1;27(9):4411-4422. doi: 10.1093/cercor/bhw243. Cereb Cortex. 2017. PMID: 27522071 Free PMC article.
References
-
- Witter MP, Groenewegen HJ, Lopes da Silva FH, Lohman AH (1989) Functional organization of the extrinsic and intrinsic circuitry of the parahippocampal region. Prog Neurobiol 33: 161–253. - PubMed
-
- Witter MP, Naber PA, van Haeften T, Machielsen WC, Rombouts SA, et al. (2000) Cortico-hippocampal communication by way of parallel parahippocampal-subicular pathways. Hippocampus 10: 398–410. - PubMed
-
- Burwell RD (2000) The parahippocampal region: corticocortical connectivity. Ann N Y Acad Sci 911: 25–42. - PubMed
-
- Steward O, Scoville SA (1976) Cells of origin of entorhinal cortical afferents to the hippocampus and fascia dentata of the rat. J Comp Neurol 169: 347–370. - PubMed
Publication types
MeSH terms
Substances
Grants and funding
LinkOut - more resources
Full Text Sources
Other Literature Sources
Miscellaneous