Novel cis-acting element within the capsid-coding region enhances flavivirus viral-RNA replication by regulating genome cyclization
- PMID: 23576500
- PMCID: PMC3676100
- DOI: 10.1128/JVI.00243-13
Novel cis-acting element within the capsid-coding region enhances flavivirus viral-RNA replication by regulating genome cyclization
Abstract
cis-Acting elements in the viral genome RNA (vRNA) are essential for the translation, replication, and/or encapsidation of RNA viruses. In this study, a novel conserved cis-acting element was identified in the capsid-coding region of mosquito-borne flavivirus. The downstream of 5' cyclization sequence (5'CS) pseudoknot (DCS-PK) element has a three-stem pseudoknot structure, as demonstrated by structure prediction and biochemical analysis. Using dengue virus as a model, we show that DCS-PK enhances vRNA replication and that its function depends on its secondary structure and specific primary sequence. Mutagenesis revealed that the highly conserved stem 1 and loop 2, which are involved in potential loop-helix interactions, are crucial for DCS-PK function. A predicted loop 1-stem 3 base triple interaction is important for the structural stability and function of DCS-PK. Moreover, the function of DCS-PK depends on its position relative to the 5'CS, and the presence of DCS-PK facilitates the formation of 5'-3' RNA complexes. Taken together, our results reveal that the cis-acting element DCS-PK enhances vRNA replication by regulating genome cyclization, and DCS-PK might interplay with other cis-acting elements to form a functional vRNA cyclization domain, thus playing critical roles during the flavivirus life cycle and evolution.
Figures
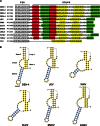
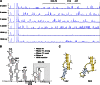
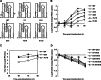
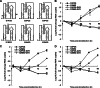
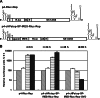
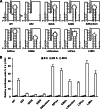
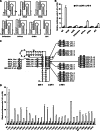
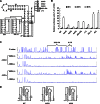
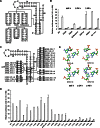
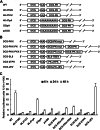
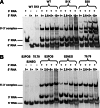
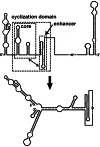
Similar articles
-
Overlapping local and long-range RNA-RNA interactions modulate dengue virus genome cyclization and replication.J Virol. 2015 Mar;89(6):3430-7. doi: 10.1128/JVI.02677-14. Epub 2015 Jan 14. J Virol. 2015. PMID: 25589642 Free PMC article.
-
Specialized cis-Acting RNA Elements Balance Genome Cyclization to Ensure Efficient Replication of Yellow Fever Virus.J Virol. 2023 Apr 27;97(4):e0194922. doi: 10.1128/jvi.01949-22. Epub 2023 Apr 5. J Virol. 2023. PMID: 37017533 Free PMC article.
-
Uncoupling cis-Acting RNA elements from coding sequences revealed a requirement of the N-terminal region of dengue virus capsid protein in virus particle formation.J Virol. 2012 Jan;86(2):1046-58. doi: 10.1128/JVI.05431-11. Epub 2011 Nov 9. J Virol. 2012. PMID: 22072762 Free PMC article.
-
Structure and function of cis-acting RNA elements of flavivirus.Rev Med Virol. 2020 Jan;30(1):e2092. doi: 10.1002/rmv.2092. Epub 2019 Nov 28. Rev Med Virol. 2020. PMID: 31777997 Review.
-
Genome cyclization as strategy for flavivirus RNA replication.Virus Res. 2009 Feb;139(2):230-9. doi: 10.1016/j.virusres.2008.07.016. Epub 2008 Sep 9. Virus Res. 2009. PMID: 18703097 Free PMC article. Review.
Cited by
-
Visualization of a neurotropic flavivirus infection in mouse reveals unique viscerotropism controlled by host type I interferon signaling.Theranostics. 2017 Feb 12;7(4):912-925. doi: 10.7150/thno.16615. eCollection 2017. Theranostics. 2017. PMID: 28382163 Free PMC article.
-
COMRADES determines in vivo RNA structures and interactions.Nat Methods. 2018 Oct;15(10):785-788. doi: 10.1038/s41592-018-0121-0. Epub 2018 Sep 10. Nat Methods. 2018. PMID: 30202058 Free PMC article.
-
RNA Structure Duplications and Flavivirus Host Adaptation.Trends Microbiol. 2016 Apr;24(4):270-283. doi: 10.1016/j.tim.2016.01.002. Epub 2016 Feb 3. Trends Microbiol. 2016. PMID: 26850219 Free PMC article. Review.
-
RanDeL-Seq: a High-Throughput Method to Map Viral cis- and trans-Acting Elements.mBio. 2021 Jan 19;12(1):e01724-20. doi: 10.1128/mBio.01724-20. mBio. 2021. PMID: 33468683 Free PMC article.
-
Using recombination-dependent lethal mutations to stabilize reporter flaviviruses for rapid serodiagnosis and drug discovery.EBioMedicine. 2020 Jul;57:102838. doi: 10.1016/j.ebiom.2020.102838. Epub 2020 Jun 20. EBioMedicine. 2020. PMID: 32574959 Free PMC article.
References
-
- Bartelma G, Padmanabhan R. 2002. Expression, purification, and characterization of the RNA 5′-triphosphatase activity of dengue virus type 2 nonstructural protein 3. Virology 299:122–132 - PubMed
-
- Benarroch D, Selisko B, Locatelli GA, Maga G, Romette JL, Canard B. 2004. The RNA helicase, nucleotide 5′-triphosphatase, and RNA 5′-triphosphatase activities of dengue virus protein NS3 are Mg2+-dependent and require a functional Walker B motif in the helicase catalytic core. Virology 328:208–218 - PubMed
Publication types
MeSH terms
Substances
LinkOut - more resources
Full Text Sources
Other Literature Sources