Genetically encoded calcium indicators for multi-color neural activity imaging and combination with optogenetics
- PMID: 23459413
- PMCID: PMC3586699
- DOI: 10.3389/fnmol.2013.00002
Genetically encoded calcium indicators for multi-color neural activity imaging and combination with optogenetics
Abstract
Genetically encoded calcium indicators (GECIs) are powerful tools for systems neuroscience. Here we describe red, single-wavelength GECIs, "RCaMPs," engineered from circular permutation of the thermostable red fluorescent protein mRuby. High-resolution crystal structures of mRuby, the red sensor RCaMP, and the recently published red GECI R-GECO1 give insight into the chromophore environments of the Ca(2+)-bound state of the sensors and the engineered protein domain interfaces of the different indicators. We characterized the biophysical properties and performance of RCaMP sensors in vitro and in vivo in Caenorhabditis elegans, Drosophila larvae, and larval zebrafish. Further, we demonstrate 2-color calcium imaging both within the same cell (registering mitochondrial and somatic [Ca(2+)]) and between two populations of cells: neurons and astrocytes. Finally, we perform integrated optogenetics experiments, wherein neural activation via channelrhodopsin-2 (ChR2) or a red-shifted variant, and activity imaging via RCaMP or GCaMP, are conducted simultaneously, with the ChR2/RCaMP pair providing independently addressable spectral channels. Using this paradigm, we measure calcium responses of naturalistic and ChR2-evoked muscle contractions in vivo in crawling C. elegans. We systematically compare the RCaMP sensors to R-GECO1, in terms of action potential-evoked fluorescence increases in neurons, photobleaching, and photoswitching. R-GECO1 displays higher Ca(2+) affinity and larger dynamic range than RCaMP, but exhibits significant photoactivation with blue and green light, suggesting that integrated channelrhodopsin-based optogenetics using R-GECO1 may be subject to artifact. Finally, we create and test blue, cyan, and yellow variants engineered from GCaMP by rational design. This engineered set of chromatic variants facilitates new experiments in functional imaging and optogenetics.
Keywords: calcium imaging; genetically encoded calcium indicator; multi-color imaging; optogenetics; protein engineering.
Figures
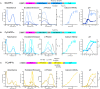
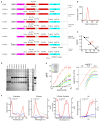
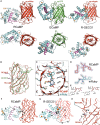
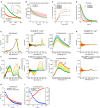
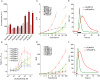
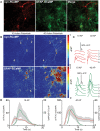
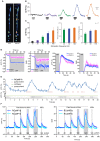
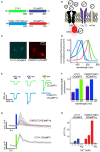
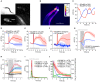
Similar articles
-
A blue-shifted genetically encoded Ca2+ indicator with enhanced two-photon absorption.bioRxiv [Preprint]. 2023 Oct 17:2023.10.12.562058. doi: 10.1101/2023.10.12.562058. bioRxiv. 2023. Update in: Neurophotonics. 2024 Apr;11(2):024207. doi: 10.1117/1.NPh.11.2.024207. PMID: 37905143 Free PMC article. Updated. Preprint.
-
Sensitive red protein calcium indicators for imaging neural activity.Elife. 2016 Mar 24;5:e12727. doi: 10.7554/eLife.12727. Elife. 2016. PMID: 27011354 Free PMC article.
-
Blue-shifted genetically encoded Ca2+ indicator with enhanced two-photon absorption.Neurophotonics. 2024 Apr;11(2):024207. doi: 10.1117/1.NPh.11.2.024207. Epub 2024 Apr 4. Neurophotonics. 2024. PMID: 38577628 Free PMC article.
-
New red-fluorescent calcium indicators for optogenetics, photoactivation and multi-color imaging.Biochim Biophys Acta. 2014 Oct;1843(10):2284-306. doi: 10.1016/j.bbamcr.2014.03.010. Epub 2014 Mar 27. Biochim Biophys Acta. 2014. PMID: 24681159 Review.
-
Fast and sensitive GCaMP calcium indicators for neuronal imaging.J Physiol. 2024 Apr;602(8):1595-1604. doi: 10.1113/JP283832. Epub 2023 Mar 14. J Physiol. 2024. PMID: 36811153 Review.
Cited by
-
Large-scale all-optical dissection of motor cortex connectivity shows a segregated organization of mouse forelimb representations.Cell Rep. 2022 Nov 8;41(6):111627. doi: 10.1016/j.celrep.2022.111627. Cell Rep. 2022. PMID: 36351410 Free PMC article.
-
Optrodes for combined optogenetics and electrophysiology in live animals.Neurophotonics. 2015 Jul;2(3):031205. doi: 10.1117/1.NPh.2.3.031205. Epub 2015 Jul 2. Neurophotonics. 2015. PMID: 26158014 Free PMC article. Review.
-
Altered activity of mPFC pyramidal neurons and parvalbumin-expressing interneurons during social interactions in a Mecp2 mouse model for Rett syndrome.bioRxiv [Preprint]. 2024 Aug 6:2024.08.06.606882. doi: 10.1101/2024.08.06.606882. bioRxiv. 2024. PMID: 39149275 Free PMC article. Preprint.
-
Supralinear Dependence of the IP3 Receptor-to-Mitochondria Local Ca2+ Transfer on the Endoplasmic Reticulum Ca2+ Loading.Contact (Thousand Oaks). 2024 Feb 14;7:25152564241229273. doi: 10.1177/25152564241229273. eCollection 2024 Jan-Dec. Contact (Thousand Oaks). 2024. PMID: 38362008 Free PMC article.
-
Subcellular ROS imaging methods: Relevance for the study of calcium signaling.Cell Calcium. 2016 Aug;60(2):65-73. doi: 10.1016/j.ceca.2016.05.001. Epub 2016 May 4. Cell Calcium. 2016. PMID: 27209367 Free PMC article. Review.
References
-
- Ai H. W., Henderson J. N., Remington S. J., Campbell R. E. (2006). Directed evolution of a monomeric, bright and photostable version of Clavularia cyan fluorescent protein: structural characterization and applications in fluorescence imaging. Biochem. J. 400, 531–540 10.1042/BJ20060874 - DOI - PMC - PubMed
-
- Akerboom J., Tian L., Marvin J. S., Looger L. L. (2012b). Engineering and application of genetically encoded calcium indicators, in Genetically Encoded Functional Indicators, ed Martin J.-R. (New York, Heidelberg, Dordrecht, London: Humana Press, Springer; ), 125–147
Grants and funding
LinkOut - more resources
Full Text Sources
Other Literature Sources
Molecular Biology Databases
Research Materials
Miscellaneous