Recurrent somatic mutation of FAT1 in multiple human cancers leads to aberrant Wnt activation
- PMID: 23354438
- PMCID: PMC3729040
- DOI: 10.1038/ng.2538
Recurrent somatic mutation of FAT1 in multiple human cancers leads to aberrant Wnt activation
Abstract
Aberrant Wnt signaling can drive cancer development. In many cancer types, the genetic basis of Wnt pathway activation remains incompletely understood. Here, we report recurrent somatic mutations of the Drosophila melanogaster tumor suppressor-related gene FAT1 in glioblastoma (20.5%), colorectal cancer (7.7%), and head and neck cancer (6.7%). FAT1 encodes a cadherin-like protein, which we found is able to potently suppress cancer cell growth in vitro and in vivo by binding β-catenin and antagonizing its nuclear localization. Inactivation of FAT1 via mutation therefore promotes Wnt signaling and tumorigenesis and affects patient survival. Taken together, these data strongly point to FAT1 as a tumor suppressor gene driving loss of chromosome 4q35, a prevalent region of deletion in cancer. Loss of FAT1 function is a frequent event during oncogenesis. These findings address two outstanding issues in cancer biology: the basis of Wnt activation in non-colorectal tumors and the identity of a 4q35 tumor suppressor.
Figures
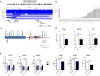
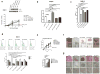
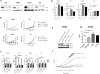
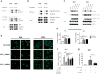
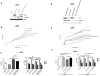
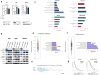
Comment in
-
The tumor suppressor FAT1 modulates WNT activity in multiple cancers.Cancer Discov. 2013 Mar;3(3):OF19. doi: 10.1158/2159-8290.CD-RW2013-031. Epub 2013 Feb 7. Cancer Discov. 2013. PMID: 23475889
Similar articles
-
APC loss-induced intestinal tumorigenesis in Drosophila: Roles of Ras in Wnt signaling activation and tumor progression.Dev Biol. 2013 Jun 15;378(2):122-40. doi: 10.1016/j.ydbio.2013.03.020. Epub 2013 Apr 6. Dev Biol. 2013. PMID: 23570874
-
Function and cancer genomics of FAT family genes (review).Int J Oncol. 2012 Dec;41(6):1913-8. doi: 10.3892/ijo.2012.1669. Epub 2012 Oct 17. Int J Oncol. 2012. PMID: 23076869 Free PMC article. Review.
-
The FAT epidemic: a gene family frequently mutated across multiple human cancer types.Cell Cycle. 2013 Apr 1;12(7):1011-2. doi: 10.4161/cc.24305. Epub 2013 Mar 14. Cell Cycle. 2013. PMID: 23493187 Free PMC article. No abstract available.
-
FAT1 expression in T-cell acute lymphoblastic leukemia (T-ALL) modulates proliferation and WNT signaling.Sci Rep. 2023 Jan 18;13(1):972. doi: 10.1038/s41598-023-27792-0. Sci Rep. 2023. PMID: 36653435 Free PMC article.
-
The diverse functions of FAT1 in cancer progression: good, bad, or ugly?J Exp Clin Cancer Res. 2022 Aug 15;41(1):248. doi: 10.1186/s13046-022-02461-8. J Exp Clin Cancer Res. 2022. PMID: 35965328 Free PMC article. Review.
Cited by
-
Recurrent somatic mutations of FAT family cadherins induce an aggressive phenotype and poor prognosis in anaplastic large cell lymphoma.Br J Cancer. 2024 Dec;131(11):1781-1795. doi: 10.1038/s41416-024-02881-7. Epub 2024 Oct 30. Br J Cancer. 2024. PMID: 39478125 Free PMC article.
-
Genomic discoveries in adult astrocytoma.Curr Opin Genet Dev. 2015 Feb;30:17-24. doi: 10.1016/j.gde.2014.12.002. Epub 2015 Jan 20. Curr Opin Genet Dev. 2015. PMID: 25616158 Free PMC article. Review.
-
Quantifying gene selection in cancer through protein functional alteration bias.Nucleic Acids Res. 2019 Jul 26;47(13):6642-6655. doi: 10.1093/nar/gkz546. Nucleic Acids Res. 2019. PMID: 31334812 Free PMC article.
-
Extracellular Matrix Alterations in Metastatic Processes.Int J Mol Sci. 2019 Oct 7;20(19):4947. doi: 10.3390/ijms20194947. Int J Mol Sci. 2019. PMID: 31591367 Free PMC article. Review.
-
A novel tankyrase inhibitor, MSC2504877, enhances the effects of clinical CDK4/6 inhibitors.Sci Rep. 2019 Jan 17;9(1):201. doi: 10.1038/s41598-018-36447-4. Sci Rep. 2019. PMID: 30655555 Free PMC article.
References
-
- Borkosky SS, et al. Frequent deletion of ING2 locus at 4q35.1 associates with advanced tumor stage in head and neck squamous cell carcinoma. J Cancer Res Clin Oncol. 2009;135:703–13. - PubMed
-
- Nakaya K, et al. Identification of homozygous deletions of tumor suppressor gene FAT in oral cancer using CGH-array. Oncogene. 2007;26:5300–8. - PubMed
Publication types
MeSH terms
Substances
Associated data
- Actions
Grants and funding
LinkOut - more resources
Full Text Sources
Other Literature Sources
Molecular Biology Databases