Quantitative fluorescence imaging reveals point of release for lipoproteins during LDLR-dependent uptake
- PMID: 23296879
- PMCID: PMC3617948
- DOI: 10.1194/jlr.M033548
Quantitative fluorescence imaging reveals point of release for lipoproteins during LDLR-dependent uptake
Abstract
The LDL receptor (LDLR) supports efficient uptake of both LDL and VLDL remnants by binding lipoprotein at the cell surface, internalizing lipoprotein through coated pits, and releasing lipoprotein in endocytic compartments before returning to the surface for further rounds of uptake. While many aspects of lipoprotein binding and receptor entry are well understood, it is less clear where, when, and how the LDLR releases lipoprotein. To address these questions, the current study employed quantitative fluorescence imaging to visualize the uptake and endosomal processing of LDL and the VLDL remnant β-VLDL. We find that lipoprotein release is rapid, with most release occurring prior to entry of lipoprotein into early endosomes. Published biochemical studies have identified two mechanisms of lipoprotein release: one that involves the β-propeller module of the LDLR and a second that is independent of this module. Quantitative imaging comparing uptake supported by the normal LDLR or by an LDLR variant incapable of β-propeller-dependent release shows that the β-propeller-independent process is sufficient for release for both lipoproteins but that the β-propeller process accelerates both LDL and β-VLDL release. Together these findings define where, when, and how lipoprotein release occurs and provide a generalizable methodology for visualizing endocytic handling in situ.
Figures
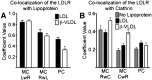
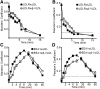
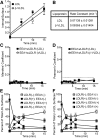
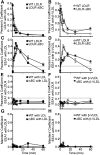
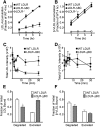
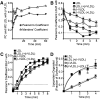
Similar articles
-
The role of calcium in lipoprotein release by the low-density lipoprotein receptor.Biochemistry. 2009 Aug 4;48(30):7313-24. doi: 10.1021/bi900214u. Biochemistry. 2009. PMID: 19583244 Free PMC article.
-
The epidermal growth factor homology domain of the LDL receptor drives lipoprotein release through an allosteric mechanism involving H190, H562, and H586.J Biol Chem. 2008 Sep 26;283(39):26528-37. doi: 10.1074/jbc.M804624200. Epub 2008 Aug 3. J Biol Chem. 2008. PMID: 18677035 Free PMC article.
-
Identification of a VLDL-induced, FDNPVY-independent internalization mechanism for the LDLR.EMBO J. 2007 Jul 25;26(14):3273-82. doi: 10.1038/sj.emboj.7601769. Epub 2007 Jun 21. EMBO J. 2007. PMID: 17581630 Free PMC article.
-
Hepatocytic lipoprotein receptors and intracellular lipoprotein catabolism.Hepatology. 1988 Nov-Dec;8(6):1689-704. doi: 10.1002/hep.1840080637. Hepatology. 1988. PMID: 2847970 Review.
-
IDL, VLDL, chylomicrons and atherosclerosis.Eur J Epidemiol. 1992 May;8 Suppl 1:92-8. doi: 10.1007/BF00145358. Eur J Epidemiol. 1992. PMID: 1505659 Review.
Cited by
-
A New Hypothesis for Alzheimer's Disease: The Lipid Invasion Model.J Alzheimers Dis Rep. 2022 Mar 25;6(1):129-161. doi: 10.3233/ADR-210299. eCollection 2022. J Alzheimers Dis Rep. 2022. PMID: 35530118 Free PMC article.
-
Targeting NPC1 in Renal Cell Carcinoma.Cancers (Basel). 2024 Jan 25;16(3):517. doi: 10.3390/cancers16030517. Cancers (Basel). 2024. PMID: 38339268 Free PMC article.
-
Identification of roles for H264, H306, H439, and H635 in acid-dependent lipoprotein release by the LDL receptor.J Lipid Res. 2017 Feb;58(2):364-374. doi: 10.1194/jlr.M070938. Epub 2016 Nov 28. J Lipid Res. 2017. PMID: 27895090 Free PMC article.
-
Cytomegalovirus immune evasion by perturbation of endosomal trafficking.Cell Mol Immunol. 2015 Mar;12(2):154-69. doi: 10.1038/cmi.2014.85. Epub 2014 Sep 29. Cell Mol Immunol. 2015. PMID: 25263490 Free PMC article. Review.
References
-
- Simonsen A., Lippe R., Christoforidis S., Gaullier J. M., Brech A., Callaghan J., Toh B. H., Murphy C., Zerial M., Stenmark H. 1998. EEA1 links PI(3)K function to Rab5 regulation of endosome fusion. Nature. 394: 494–498 - PubMed
-
- Mills I. G., Jones A. T., Clague M. J. 1999. Regulation of endosome fusion. Mol. Membr. Biol. 16: 73–79 - PubMed
-
- Tycko B., Maxfield F. R. 1982. Rapid acidification of endocytic vesicles containing alpha 2-macroglobulin. Cell. 28: 643–651 - PubMed
-
- Gerasimenko J. V., Tepikin A. V., Petersen O. H., Gerasimenko O. V. 1998. Calcium uptake via endocytosis with rapid release from acidifying endosomes. Curr. Biol. 8: 1335–1338 - PubMed
Publication types
MeSH terms
Substances
Grants and funding
LinkOut - more resources
Full Text Sources
Other Literature Sources