Architecture of the Atg17 complex as a scaffold for autophagosome biogenesis
- PMID: 23219485
- PMCID: PMC3806636
- DOI: 10.1016/j.cell.2012.11.028
Architecture of the Atg17 complex as a scaffold for autophagosome biogenesis
Abstract
Macroautophagy is a bulk clearance mechanism in which the double-membraned phagophore grows and engulfs cytosolic material. In yeast, the phagophore nucleates from a cluster of 20-30 nm diameter Atg9-containing vesicles located at a multiprotein assembly known as the preautophagosomal structure (PAS). The crystal structure of a 2:2:2 complex of the earliest acting PAS proteins, Atg17, Atg29, and Atg31, was solved at 3.05 Å resolution. Atg17 is crescent shaped with a 10 nm radius of curvature. Dimerization of the Atg17-Atg31-Atg29 complex is critical for both PAS formation and autophagy, and each dimer contains two separate and complete crescents. Upon induction of autophagy, Atg17-Atg31-Atg29 assembles with Atg1 and Atg13, which in turn initiates the formation of the phagophore. The C-terminal EAT domain of Atg1 was shown to sense membrane curvature, dimerize, and tether lipid vesicles. These data suggest a structural mechanism for the organization of Atg9 vesicles into the early phagophore.
Copyright © 2012 Elsevier Inc. All rights reserved.
Figures
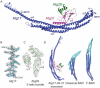
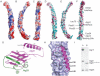
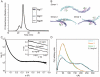
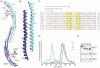
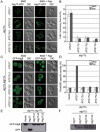
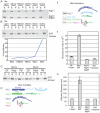
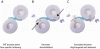
Comment in
-
A dimer to bridge early autophagosomal membranes.Cell. 2012 Dec 21;151(7):1403-5. doi: 10.1016/j.cell.2012.12.008. Cell. 2012. PMID: 23260133
Similar articles
-
Molecular interactions of the Saccharomyces cerevisiae Atg1 complex provide insights into assembly and regulatory mechanisms.Autophagy. 2015;11(6):891-905. doi: 10.1080/15548627.2015.1040972. Autophagy. 2015. PMID: 25998554 Free PMC article.
-
Solution structure of the Atg1 complex: implications for the architecture of the phagophore assembly site.Structure. 2015 May 5;23(5):809-818. doi: 10.1016/j.str.2015.02.012. Epub 2015 Mar 26. Structure. 2015. PMID: 25817386 Free PMC article.
-
The role of Atg29 phosphorylation in PAS assembly.Autophagy. 2013 Dec;9(12):2178-9. doi: 10.4161/auto.26740. Epub 2013 Oct 16. Autophagy. 2013. PMID: 24141181 Free PMC article.
-
The Atg17-Atg31-Atg29 Complex Coordinates with Atg11 to Recruit the Vam7 SNARE and Mediate Autophagosome-Vacuole Fusion.Curr Biol. 2016 Jan 25;26(2):150-160. doi: 10.1016/j.cub.2015.11.054. Epub 2016 Jan 7. Curr Biol. 2016. PMID: 26774783 Free PMC article. Review.
-
The beginning of the end: how scaffolds nucleate autophagosome biogenesis.Trends Cell Biol. 2014 Jan;24(1):73-81. doi: 10.1016/j.tcb.2013.07.008. Epub 2013 Aug 30. Trends Cell Biol. 2014. PMID: 23999079 Free PMC article. Review.
Cited by
-
Palmitoylation of ULK1 by ZDHHC13 plays a crucial role in autophagy.Nat Commun. 2024 Aug 21;15(1):7194. doi: 10.1038/s41467-024-51402-w. Nat Commun. 2024. PMID: 39169022 Free PMC article.
-
Atg1 family kinases in autophagy initiation.Cell Mol Life Sci. 2015 Aug;72(16):3083-96. doi: 10.1007/s00018-015-1917-z. Epub 2015 May 7. Cell Mol Life Sci. 2015. PMID: 25948417 Free PMC article. Review.
-
ER exit sites are physical and functional core autophagosome biogenesis components.Mol Biol Cell. 2013 Sep;24(18):2918-31. doi: 10.1091/mbc.E13-07-0381. Epub 2013 Jul 31. Mol Biol Cell. 2013. PMID: 23904270 Free PMC article.
-
The Multivesicular Body and Autophagosome Pathways in Plants.Front Plant Sci. 2018 Dec 12;9:1837. doi: 10.3389/fpls.2018.01837. eCollection 2018. Front Plant Sci. 2018. PMID: 30619408 Free PMC article.
-
Theater in the Self-Cleaning Cell: Intrinsically Disordered Proteins or Protein Regions Acting with Membranes in Autophagy.Membranes (Basel). 2022 Apr 24;12(5):457. doi: 10.3390/membranes12050457. Membranes (Basel). 2022. PMID: 35629783 Free PMC article. Review.
References
-
- Choi JD, Ryu M, Ae Park M, Jeong G, Lee JS. FIP200 inhibits beta-catenin-mediated transcription by promoting APC-independent beta-catenin ubiquitination. Oncogene. 2012 epub ahead of print. - PubMed
-
- Cowtan K, Main P. Miscellaneous algorithms for density modification. Acta Crystallogr Sect D-Biol Crystallogr. 1998;54:487–493. - PubMed
Publication types
MeSH terms
Substances
Associated data
- Actions
Grants and funding
LinkOut - more resources
Full Text Sources
Other Literature Sources
Molecular Biology Databases
Research Materials