Optimization of a GCaMP calcium indicator for neural activity imaging
- PMID: 23035093
- PMCID: PMC3482105
- DOI: 10.1523/JNEUROSCI.2601-12.2012
Optimization of a GCaMP calcium indicator for neural activity imaging
Abstract
Genetically encoded calcium indicators (GECIs) are powerful tools for systems neuroscience. Recent efforts in protein engineering have significantly increased the performance of GECIs. The state-of-the art single-wavelength GECI, GCaMP3, has been deployed in a number of model organisms and can reliably detect three or more action potentials in short bursts in several systems in vivo. Through protein structure determination, targeted mutagenesis, high-throughput screening, and a battery of in vitro assays, we have increased the dynamic range of GCaMP3 by severalfold, creating a family of "GCaMP5" sensors. We tested GCaMP5s in several systems: cultured neurons and astrocytes, mouse retina, and in vivo in Caenorhabditis chemosensory neurons, Drosophila larval neuromuscular junction and adult antennal lobe, zebrafish retina and tectum, and mouse visual cortex. Signal-to-noise ratio was improved by at least 2- to 3-fold. In the visual cortex, two GCaMP5 variants detected twice as many visual stimulus-responsive cells as GCaMP3. By combining in vivo imaging with electrophysiology we show that GCaMP5 fluorescence provides a more reliable measure of neuronal activity than its predecessor GCaMP3. GCaMP5 allows more sensitive detection of neural activity in vivo and may find widespread applications for cellular imaging in general.
Figures
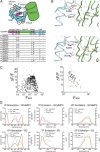
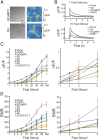
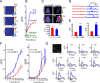
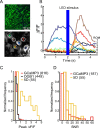


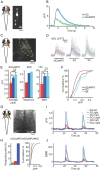
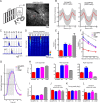
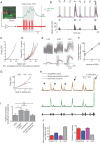
Similar articles
-
Imaging neural activity in worms, flies and mice with improved GCaMP calcium indicators.Nat Methods. 2009 Dec;6(12):875-81. doi: 10.1038/nmeth.1398. Epub 2009 Nov 8. Nat Methods. 2009. PMID: 19898485 Free PMC article.
-
Green-to-Red Photoconversion of GCaMP.PLoS One. 2015 Sep 18;10(9):e0138127. doi: 10.1371/journal.pone.0138127. eCollection 2015. PLoS One. 2015. PMID: 26382605 Free PMC article.
-
Functional imaging in the zebrafish retinotectal system using RGECO.Front Neural Circuits. 2013 Mar 6;7:34. doi: 10.3389/fncir.2013.00034. eCollection 2013. Front Neural Circuits. 2013. PMID: 23508811 Free PMC article.
-
Targeted optical probing of neuronal circuit dynamics using fluorescent protein sensors.Neurosignals. 2008;16(4):289-99. doi: 10.1159/000123039. Epub 2008 Jul 18. Neurosignals. 2008. PMID: 18635945 Review.
-
Structure- and mechanism-guided design of single fluorescent protein-based biosensors.Nat Chem Biol. 2021 May;17(5):509-518. doi: 10.1038/s41589-020-00718-x. Epub 2021 Feb 8. Nat Chem Biol. 2021. PMID: 33558715 Review.
Cited by
-
Automated quantification of neuronal networks and single-cell calcium dynamics using calcium imaging.J Neurosci Methods. 2015 Mar 30;243:26-38. doi: 10.1016/j.jneumeth.2015.01.020. Epub 2015 Jan 25. J Neurosci Methods. 2015. PMID: 25629800 Free PMC article.
-
Ex Vivo Analysis of Mechanically Activated Ca2+ Transients in Urothelial Cells.J Vis Exp. 2022 Sep 28;(187):10.3791/64532. doi: 10.3791/64532. J Vis Exp. 2022. PMID: 36279534 Free PMC article.
-
Rapid single-step induction of functional neurons from human pluripotent stem cells.Neuron. 2013 Jun 5;78(5):785-98. doi: 10.1016/j.neuron.2013.05.029. Neuron. 2013. PMID: 23764284 Free PMC article.
-
Pixels as ROIs (PAR): a less-biased and statistically powerful approach for gleaning functional information from image stacks.PLoS One. 2013 Jul 11;8(7):e69047. doi: 10.1371/journal.pone.0069047. Print 2013. PLoS One. 2013. PMID: 23874862 Free PMC article.
-
Lights, fiber, action! A primer on in vivo fiber photometry.Neuron. 2024 Mar 6;112(5):718-739. doi: 10.1016/j.neuron.2023.11.016. Epub 2023 Dec 15. Neuron. 2024. PMID: 38103545 Free PMC article. Review.
References
Publication types
MeSH terms
Substances
Grants and funding
LinkOut - more resources
Full Text Sources
Other Literature Sources
Molecular Biology Databases
Research Materials