A paravascular pathway facilitates CSF flow through the brain parenchyma and the clearance of interstitial solutes, including amyloid β
- PMID: 22896675
- PMCID: PMC3551275
- DOI: 10.1126/scitranslmed.3003748
A paravascular pathway facilitates CSF flow through the brain parenchyma and the clearance of interstitial solutes, including amyloid β
Abstract
Because it lacks a lymphatic circulation, the brain must clear extracellular proteins by an alternative mechanism. The cerebrospinal fluid (CSF) functions as a sink for brain extracellular solutes, but it is not clear how solutes from the brain interstitium move from the parenchyma to the CSF. We demonstrate that a substantial portion of subarachnoid CSF cycles through the brain interstitial space. On the basis of in vivo two-photon imaging of small fluorescent tracers, we showed that CSF enters the parenchyma along paravascular spaces that surround penetrating arteries and that brain interstitial fluid is cleared along paravenous drainage pathways. Animals lacking the water channel aquaporin-4 (AQP4) in astrocytes exhibit slowed CSF influx through this system and a ~70% reduction in interstitial solute clearance, suggesting that the bulk fluid flow between these anatomical influx and efflux routes is supported by astrocytic water transport. Fluorescent-tagged amyloid β, a peptide thought to be pathogenic in Alzheimer's disease, was transported along this route, and deletion of the Aqp4 gene suppressed the clearance of soluble amyloid β, suggesting that this pathway may remove amyloid β from the central nervous system. Clearance through paravenous flow may also regulate extracellular levels of proteins involved with neurodegenerative conditions, its impairment perhaps contributing to the mis-accumulation of soluble proteins.
Conflict of interest statement
Figures
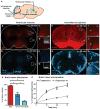
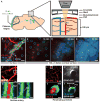
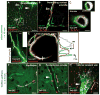
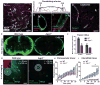
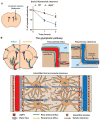
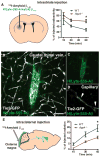
Comment in
-
Paravascular Pathways in the Eye: Is There an 'Ocular Glymphatic System'?Invest Ophthalmol Vis Sci. 2015 Jun;56(6):3955-6. doi: 10.1167/iovs.15-17243. Invest Ophthalmol Vis Sci. 2015. PMID: 26087361 No abstract available.
Similar articles
-
Cerebral arterial pulsation drives paravascular CSF-interstitial fluid exchange in the murine brain.J Neurosci. 2013 Nov 13;33(46):18190-9. doi: 10.1523/JNEUROSCI.1592-13.2013. J Neurosci. 2013. PMID: 24227727 Free PMC article.
-
Impairment of paravascular clearance pathways in the aging brain.Ann Neurol. 2014 Dec;76(6):845-61. doi: 10.1002/ana.24271. Epub 2014 Sep 26. Ann Neurol. 2014. PMID: 25204284 Free PMC article.
-
Clearance from the mouse brain by convection of interstitial fluid towards the ventricular system.Fluids Barriers CNS. 2015 Oct 5;12:23. doi: 10.1186/s12987-015-0019-5. Fluids Barriers CNS. 2015. PMID: 26435380 Free PMC article.
-
The role of brain barriers in fluid movement in the CNS: is there a 'glymphatic' system?Acta Neuropathol. 2018 Mar;135(3):387-407. doi: 10.1007/s00401-018-1812-4. Epub 2018 Feb 10. Acta Neuropathol. 2018. PMID: 29428972 Review.
-
The Paravascular Pathway for Brain Waste Clearance: Current Understanding, Significance and Controversy.Front Neuroanat. 2017 Nov 7;11:101. doi: 10.3389/fnana.2017.00101. eCollection 2017. Front Neuroanat. 2017. PMID: 29163074 Free PMC article. Review.
Cited by
-
Diffusion of macromolecules in the brain: implications for drug delivery.Mol Pharm. 2013 May 6;10(5):1492-504. doi: 10.1021/mp300495e. Epub 2013 Jan 31. Mol Pharm. 2013. PMID: 23298378 Free PMC article. Review.
-
Challenges and Future Perspectives in Modeling Neurodegenerative Diseases Using Organ-on-a-Chip Technology.Adv Sci (Weinh). 2024 Aug;11(32):e2403892. doi: 10.1002/advs.202403892. Epub 2024 Jun 23. Adv Sci (Weinh). 2024. PMID: 38922799 Free PMC article. Review.
-
Healthy young hearts sharper older minds make.Ann Neurol. 2013 Feb;73(2):151-2. doi: 10.1002/ana.23847. Ann Neurol. 2013. PMID: 23526555 Free PMC article. No abstract available.
-
Impaired glymphatic function as a biomarker for subjective cognitive decline: An exploratory dual cohort study.Alzheimers Dement. 2024 Sep;20(9):6542-6555. doi: 10.1002/alz.14149. Epub 2024 Aug 6. Alzheimers Dement. 2024. PMID: 39107995 Free PMC article.
-
Maternal and infant factors associated with infancy-onset hydrocephalus in Washington State.Pediatr Neurol. 2015 Mar;52(3):320-5. doi: 10.1016/j.pediatrneurol.2014.10.030. Epub 2014 Nov 10. Pediatr Neurol. 2015. PMID: 25542767 Free PMC article.
References
-
- Aukland K, Reed RK. Interstitial-lymphatic mechanisms in the control of extracellular fluid volume. Physiol Rev. 1993;73:1–78. - PubMed
-
- Schmid-Schönbein GW. Microlymphatics and lymph flow. Physiol Rev. 1990;70:987–1028. - PubMed
-
- Abbott NJ. Evidence for bulk flow of brain interstitial fluid: Significance for physiology and pathology. Neurochem Int. 2004;45:545–552. - PubMed
-
- Cserr HF, Cooper DN, Suri PK, Patlak CS. Efflux of radiolabeled polyethylene glycols and albumin from rat brain. Am J Physiol. 1981;240:F319–F328. - PubMed
-
- Cserr HF, Harling-Berg CJ, Knopf PM. Drainage of brain extracellular fluid into blood and deep cervical lymph and its immunological significance. Brain Pathol. 1992;2:269–276. - PubMed
Publication types
MeSH terms
Substances
Grants and funding
LinkOut - more resources
Full Text Sources
Other Literature Sources
Molecular Biology Databases