Oxidative phosphorylation, not glycolysis, powers presynaptic and postsynaptic mechanisms underlying brain information processing
- PMID: 22745494
- PMCID: PMC3390246
- DOI: 10.1523/JNEUROSCI.0026-12.2012
Oxidative phosphorylation, not glycolysis, powers presynaptic and postsynaptic mechanisms underlying brain information processing
Abstract
Neural activity has been suggested to initially trigger ATP production by glycolysis, rather than oxidative phosphorylation, for three reasons: glycolytic enzymes are associated with ion pumps; neurons may increase their energy supply by activating glycolysis in astrocytes to generate lactate; and activity increases glucose uptake more than O₂ uptake. In rat hippocampal slices, neuronal activity rapidly decreased the levels of extracellular O₂ and intracellular NADH (reduced nicotinamide adenine dinucleotide), even with lactate dehydrogenase blocked to prevent lactate generation, or with only 20% superfused O₂ to mimic physiological O₂ levels. Pharmacological analysis revealed an energy budget in which 11% of O₂ use was on presynaptic action potentials, 17% was on presynaptic Ca²⁺ entry and transmitter release, 46% was on postsynaptic glutamate receptors, and 26% was on postsynaptic action potentials, in approximate accord with theoretical brain energy budgets. Thus, the major mechanisms mediating brain information processing are all initially powered by oxidative phosphorylation, and an astrocyte-neuron lactate shuttle is not needed for this to occur.
Figures
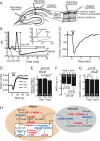
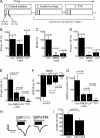
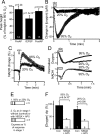
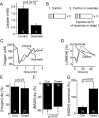
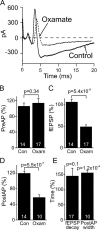
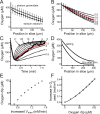
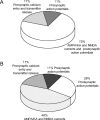
Similar articles
-
Presynaptic α4β2 nicotinic acetylcholine receptors increase glutamate release and serotonin neuron excitability in the dorsal raphe nucleus.J Neurosci. 2012 Oct 24;32(43):15148-57. doi: 10.1523/JNEUROSCI.0941-12.2012. J Neurosci. 2012. PMID: 23100436 Free PMC article.
-
Distinct mechanisms of presynaptic inhibition at GABAergic synapses of the rat substantia nigra pars compacta.J Neurophysiol. 2005 Sep;94(3):1992-2003. doi: 10.1152/jn.00171.2005. Epub 2005 Jun 8. J Neurophysiol. 2005. PMID: 15944237
-
Pregnenolone sulfate enhances spontaneous glutamate release by inducing presynaptic Ca2+-induced Ca2+ release.Neuroscience. 2010 Nov 24;171(1):106-16. doi: 10.1016/j.neuroscience.2010.07.057. Epub 2010 Sep 17. Neuroscience. 2010. PMID: 20816925
-
Modulation of allopregnanolone on excitatory transmitters release from single glutamatergic terminal.Brain Res Bull. 2013 Apr;93:39-46. doi: 10.1016/j.brainresbull.2012.11.002. Epub 2012 Nov 19. Brain Res Bull. 2013. PMID: 23174309 Review.
-
Lactate as a supplemental fuel for synaptic transmission and neuronal network oscillations: Potentials and limitations.J Neurochem. 2024 May;168(5):608-631. doi: 10.1111/jnc.15867. Epub 2023 Jun 13. J Neurochem. 2024. PMID: 37309602 Review.
Cited by
-
Oxidative stress and Parkinson's disease.Front Neuroanat. 2015 Jul 8;9:91. doi: 10.3389/fnana.2015.00091. eCollection 2015. Front Neuroanat. 2015. PMID: 26217195 Free PMC article. Review.
-
Glycolysis selectively shapes the presynaptic action potential waveform.J Neurophysiol. 2016 Dec 1;116(6):2523-2540. doi: 10.1152/jn.00629.2016. Epub 2016 Sep 7. J Neurophysiol. 2016. PMID: 27605535 Free PMC article.
-
Postsynaptic mitochondria are positioned to support functional diversity of dendritic spines.Elife. 2023 Dec 7;12:RP89682. doi: 10.7554/eLife.89682. Elife. 2023. PMID: 38059805 Free PMC article.
-
A Ca2+-Dependent Mechanism Boosting Glycolysis and OXPHOS by Activating Aralar-Malate-Aspartate Shuttle, upon Neuronal Stimulation.J Neurosci. 2022 May 11;42(19):3879-3895. doi: 10.1523/JNEUROSCI.1463-21.2022. Epub 2022 Apr 6. J Neurosci. 2022. PMID: 35387872 Free PMC article.
-
Brain capillary pericytes are metabolic sentinels that control blood flow through a KATP channel-dependent energy switch.Cell Rep. 2022 Dec 27;41(13):111872. doi: 10.1016/j.celrep.2022.111872. Cell Rep. 2022. PMID: 36577387 Free PMC article.
References
-
- Ali MA, Yasui F, Matsugo S, Konishi T. The lactate-dependent enhancement of hydroxyl radical generation by the Fenton reaction. Free Radic Res. 2000;32:429–438. - PubMed
-
- Alle H, Roth A, Geiger JR. Energy-efficient action potentials in hippocampal mossy fibers. Science. 2009;325:1405–1408. - PubMed
-
- Amato A, Ballerini L, Attwell D. Intracellular pH changes produced by glutamate uptake in rat hippocampal slices. J Neurophysiol. 1994;72:1686–1696. - PubMed
-
- Attwell D, Laughlin SB. An energy budget for signaling in the grey matter of the brain. J Cereb Blood Flow Metab. 2001;21:1133–1145. - PubMed
Publication types
MeSH terms
Substances
Grants and funding
LinkOut - more resources
Full Text Sources
Miscellaneous