Structural and biochemical consequences of disease-causing mutations in the ankyrin repeat domain of the human TRPV4 channel
- PMID: 22702953
- PMCID: PMC3413242
- DOI: 10.1021/bi300279b
Structural and biochemical consequences of disease-causing mutations in the ankyrin repeat domain of the human TRPV4 channel
Abstract
The TRPV4 calcium-permeable cation channel plays important physiological roles in osmosensation, mechanosensation, cell barrier formation, and bone homeostasis. Recent studies reported that mutations in TRPV4, including some in its ankyrin repeat domain (ARD), are associated with human inherited diseases, including neuropathies and skeletal dysplasias, probably because of the increased constitutive activity of the channel. TRPV4 activity is regulated by the binding of calmodulin and small molecules such as ATP to the ARD at its cytoplasmic N-terminus. We determined structures of ATP-free and -bound forms of human TRPV4-ARD and compared them with available TRPV-ARD structures. The third inter-repeat loop region (Finger 3 loop) is flexible and may act as a switch to regulate channel activity. Comparisons of TRPV-ARD structures also suggest an evolutionary link between ARD structure and ATP binding ability. Thermal stability analyses and molecular dynamics simulations suggest that ATP increases stability in TRPV-ARDs that can bind ATP. Biochemical analyses of a large panel of TRPV4-ARD mutations associated with human inherited diseases showed that some impaired thermal stability while others weakened ATP binding ability, suggesting molecular mechanisms for the diseases.
Figures
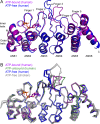
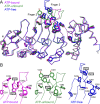
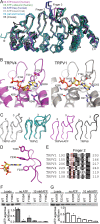
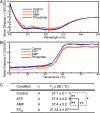
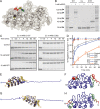
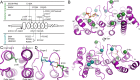
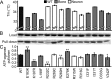
Similar articles
-
The structural changes of the mutated ankyrin repeat domain of the human TRPV4 channel alter its ATP binding ability.J Mech Behav Biomed Mater. 2020 Jan;101:103407. doi: 10.1016/j.jmbbm.2019.103407. Epub 2019 Aug 28. J Mech Behav Biomed Mater. 2020. PMID: 31493693
-
Structural analyses of the ankyrin repeat domain of TRPV6 and related TRPV ion channels.Biochemistry. 2008 Feb 26;47(8):2476-84. doi: 10.1021/bi702109w. Epub 2008 Jan 31. Biochemistry. 2008. PMID: 18232717 Free PMC article.
-
TRPV4 channel activity is modulated by direct interaction of the ankyrin domain to PI(4,5)P₂.Nat Commun. 2014 Sep 26;5:4994. doi: 10.1038/ncomms5994. Nat Commun. 2014. PMID: 25256292
-
TRPV4-pathy, a novel channelopathy affecting diverse systems.J Hum Genet. 2010 Jul;55(7):400-2. doi: 10.1038/jhg.2010.37. Epub 2010 May 27. J Hum Genet. 2010. PMID: 20505684 Review.
-
Modulation of TRPV4 by diverse mechanisms.Int J Biochem Cell Biol. 2016 Sep;78:217-228. doi: 10.1016/j.biocel.2016.07.012. Epub 2016 Jul 15. Int J Biochem Cell Biol. 2016. PMID: 27425399 Review.
Cited by
-
Evolution and folding of repeat proteins.Proc Natl Acad Sci U S A. 2022 Aug 2;119(31):e2204131119. doi: 10.1073/pnas.2204131119. Epub 2022 Jul 29. Proc Natl Acad Sci U S A. 2022. PMID: 35905321 Free PMC article.
-
Novel mutations highlight the key role of the ankyrin repeat domain in TRPV4-mediated neuropathy.Neurol Genet. 2015 Oct 22;1(4):e29. doi: 10.1212/NXG.0000000000000029. eCollection 2015 Dec. Neurol Genet. 2015. PMID: 27066566 Free PMC article.
-
Neuropathy-causing TRPV4 mutations disrupt TRPV4-RhoA interactions and impair neurite extension.Nat Commun. 2021 Mar 4;12(1):1444. doi: 10.1038/s41467-021-21699-y. Nat Commun. 2021. PMID: 33664271 Free PMC article.
-
Phosphatidylinositol-4,5-biphosphate-dependent rearrangement of TRPV4 cytosolic tails enables channel activation by physiological stimuli.Proc Natl Acad Sci U S A. 2013 Jun 4;110(23):9553-8. doi: 10.1073/pnas.1220231110. Epub 2013 May 20. Proc Natl Acad Sci U S A. 2013. PMID: 23690576 Free PMC article.
-
Human skeletal dysplasia caused by a constitutive activated transient receptor potential vanilloid 4 (TRPV4) cation channel mutation.Exp Mol Med. 2012 Dec 31;44(12):707-22. doi: 10.3858/emm.2012.44.12.080. Exp Mol Med. 2012. PMID: 23143559 Free PMC article. Review.
References
-
- Clapham D. E. (2003) TRP channels as cellular sensors. Nature 426, 517–524. - PubMed
-
- Clapham D. E.; Montell C.; Schultz G.; Julius D. (2003) International Union of Pharmacology. XLIII. Compendium of voltage-gated ion channels: Transient receptor potential channels. Pharmacol. Rev. 55, 591–596. - PubMed
-
- Tominaga M.; Tominaga T. (2005) Structure and function of TRPV1. Pfluegers Arch. 451, 143–150. - PubMed
-
- van de Graaf S. F.; Hoenderop J. G.; Bindels R. J. (2006) Regulation of TRPV5 and TRPV6 by associated proteins. Am. J. Physiol. 290, F1295–F1302. - PubMed
Publication types
MeSH terms
Substances
Grants and funding
LinkOut - more resources
Full Text Sources
Other Literature Sources
Molecular Biology Databases