FMRP stalls ribosomal translocation on mRNAs linked to synaptic function and autism
- PMID: 21784246
- PMCID: PMC3232425
- DOI: 10.1016/j.cell.2011.06.013
FMRP stalls ribosomal translocation on mRNAs linked to synaptic function and autism
Abstract
FMRP loss of function causes Fragile X syndrome (FXS) and autistic features. FMRP is a polyribosome-associated neuronal RNA-binding protein, suggesting that it plays a key role in regulating neuronal translation, but there has been little consensus regarding either its RNA targets or mechanism of action. Here, we use high-throughput sequencing of RNAs isolated by crosslinking immunoprecipitation (HITS-CLIP) to identify FMRP interactions with mouse brain polyribosomal mRNAs. FMRP interacts with the coding region of transcripts encoding pre- and postsynaptic proteins and transcripts implicated in autism spectrum disorders (ASD). We developed a brain polyribosome-programmed translation system, revealing that FMRP reversibly stalls ribosomes specifically on its target mRNAs. Our results suggest that loss of a translational brake on the synthesis of a subset of synaptic proteins contributes to FXS. In addition, they provide insight into the molecular basis of the cognitive and allied defects in FXS and ASD and suggest multiple targets for clinical intervention.
Copyright © 2011 Elsevier Inc. All rights reserved.
Conflict of interest statement
The authors declare no competing financial interests.
Figures
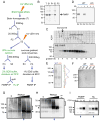
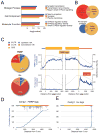
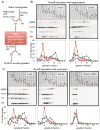
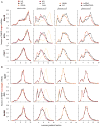
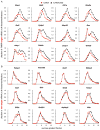
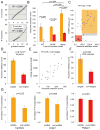
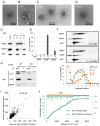
Similar articles
-
FMRP has a cell-type-specific role in CA1 pyramidal neurons to regulate autism-related transcripts and circadian memory.Elife. 2019 Dec 20;8:e46919. doi: 10.7554/eLife.46919. Elife. 2019. PMID: 31860442 Free PMC article.
-
The translation of translational control by FMRP: therapeutic targets for FXS.Nat Neurosci. 2013 Nov;16(11):1530-6. doi: 10.1038/nn.3379. Epub 2013 Apr 14. Nat Neurosci. 2013. PMID: 23584741 Free PMC article. Review.
-
The state of synapses in fragile X syndrome.Neuroscientist. 2009 Oct;15(5):549-67. doi: 10.1177/1073858409333075. Epub 2009 Mar 26. Neuroscientist. 2009. PMID: 19325170 Free PMC article. Review.
-
Molecular and cellular aspects of mental retardation in the Fragile X syndrome: from gene mutation/s to spine dysmorphogenesis.Adv Exp Med Biol. 2012;970:517-51. doi: 10.1007/978-3-7091-0932-8_23. Adv Exp Med Biol. 2012. PMID: 22351071 Review.
-
Pumping the brakes: A noncanonical RNA-binding domain in FMRP stalls elongating ribosomes.J Biol Chem. 2023 Jan;299(1):102773. doi: 10.1016/j.jbc.2022.102773. Epub 2022 Dec 5. J Biol Chem. 2023. PMID: 36481269 Free PMC article.
Cited by
-
Homeostatic responses fail to correct defective amygdala inhibitory circuit maturation in fragile X syndrome.J Neurosci. 2013 Apr 24;33(17):7548-58. doi: 10.1523/JNEUROSCI.2764-12.2013. J Neurosci. 2013. PMID: 23616559 Free PMC article.
-
Phosphorylation of FMRP and alterations of FMRP complex underlie enhanced mLTD in adult rats triggered by early life seizures.Neurobiol Dis. 2013 Nov;59:1-17. doi: 10.1016/j.nbd.2013.06.013. Epub 2013 Jul 2. Neurobiol Dis. 2013. PMID: 23831253 Free PMC article.
-
m6A readers, writers, erasers, and the m6A epitranscriptome in breast cancer.J Mol Endocrinol. 2022 Dec 21;70(2):e220110. doi: 10.1530/JME-22-0110. Print 2023 Feb 1. J Mol Endocrinol. 2022. PMID: 36367225 Free PMC article. Review.
-
Mnk1/2 kinases regulate memory and autism-related behaviours via Syngap1.Brain. 2023 May 2;146(5):2175-2190. doi: 10.1093/brain/awac398. Brain. 2023. PMID: 36315645 Free PMC article.
-
Regulation of monocyte induced cell migration by the RNA binding protein, FXR1.Cell Cycle. 2016 Jul 17;15(14):1874-82. doi: 10.1080/15384101.2016.1189040. Epub 2016 May 26. Cell Cycle. 2016. PMID: 27229378 Free PMC article.
References
Publication types
MeSH terms
Substances
Associated data
- Actions
Grants and funding
LinkOut - more resources
Full Text Sources
Other Literature Sources
Medical
Molecular Biology Databases
Miscellaneous