Nonspecifically bound proteins spin while diffusing along DNA
- PMID: 19898474
- PMCID: PMC2889498
- DOI: 10.1038/nsmb.1716
Nonspecifically bound proteins spin while diffusing along DNA
Abstract
It is known that DNA-binding proteins can slide along the DNA helix while searching for specific binding sites, but their path of motion remains obscure. Do these proteins undergo simple one-dimensional (1D) translational diffusion, or do they rotate to maintain a specific orientation with respect to the DNA helix? We measured 1D diffusion constants as a function of protein size while maintaining the DNA-protein interface. Using bootstrap analysis of single-molecule diffusion data, we compared the results to theoretical predictions for pure translational motion and rotation-coupled sliding along the DNA. The data indicate that DNA-binding proteins undergo rotation-coupled sliding along the DNA helix and can be described by a model of diffusion along the DNA helix on a rugged free-energy landscape. A similar analysis including the 1D diffusion constants of eight proteins of varying size shows that rotation-coupled sliding is a general phenomenon. The average free-energy barrier for sliding along the DNA was 1.1 +/- 0.2 k(B)T. Such small barriers facilitate rapid search for binding sites.
Figures
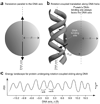
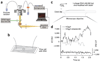
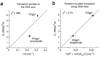
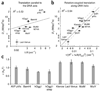
Similar articles
-
High Free-Energy Barrier of 1D Diffusion Along DNA by Architectural DNA-Binding Proteins.J Mol Biol. 2018 Mar 2;430(5):655-667. doi: 10.1016/j.jmb.2018.01.001. Epub 2018 Jan 4. J Mol Biol. 2018. PMID: 29307468 Free PMC article.
-
Weak frustration regulates sliding and binding kinetics on rugged protein-DNA landscapes.J Phys Chem B. 2013 Oct 24;117(42):13005-14. doi: 10.1021/jp402296d. Epub 2013 May 24. J Phys Chem B. 2013. PMID: 23668488
-
Diffusion constant of a nonspecifically bound protein undergoing curvilinear motion along DNA.J Phys Chem B. 2008 May 15;112(19):6282-4. doi: 10.1021/jp077568f. Epub 2008 Mar 6. J Phys Chem B. 2008. PMID: 18321088
-
An end to 40 years of mistakes in DNA-protein association kinetics?Biochem Soc Trans. 2009 Apr;37(Pt 2):343-8. doi: 10.1042/BST0370343. Biochem Soc Trans. 2009. PMID: 19290859 Review.
-
Intrinsically disordered regions as affinity tuners in protein-DNA interactions.Mol Biosyst. 2012 Jan;8(1):47-57. doi: 10.1039/c1mb05273j. Epub 2011 Sep 15. Mol Biosyst. 2012. PMID: 21918774 Review.
Cited by
-
ATP alters the diffusion mechanics of MutS on mismatched DNA.Structure. 2012 Jul 3;20(7):1264-1274. doi: 10.1016/j.str.2012.04.017. Epub 2012 Jun 7. Structure. 2012. PMID: 22682745 Free PMC article.
-
Regulation of a viral proteinase by a peptide and DNA in one-dimensional space: II. adenovirus proteinase is activated in an unusual one-dimensional biochemical reaction.J Biol Chem. 2013 Jan 18;288(3):2068-80. doi: 10.1074/jbc.M112.407312. Epub 2012 Oct 7. J Biol Chem. 2013. PMID: 23043137 Free PMC article.
-
Retroviral intasomes search for a target DNA by 1D diffusion which rarely results in integration.Nat Commun. 2016 Apr 25;7:11409. doi: 10.1038/ncomms11409. Nat Commun. 2016. PMID: 27108531 Free PMC article.
-
MutS switches between two fundamentally distinct clamps during mismatch repair.Nat Struct Mol Biol. 2011 Mar;18(3):379-85. doi: 10.1038/nsmb.2009. Epub 2011 Jan 30. Nat Struct Mol Biol. 2011. PMID: 21278758 Free PMC article.
-
Positive and negative design for nonconsensus protein-DNA binding affinity in the vicinity of functional binding sites.Biophys J. 2013 Oct 1;105(7):1653-60. doi: 10.1016/j.bpj.2013.08.033. Biophys J. 2013. PMID: 24094406 Free PMC article.
References
-
- Berg OG, Winter RB. Von Hippel, P.H. Diffusion-driven mechanisms of protein translocation on nucleic acids. 1. Models and theory. Biochemistry. 1981;20:6929–6948. - PubMed
-
- Von Hippel PH, Berg OG. Facilitated target location in biological systems. J. Biol. Chem. 1989;264:675–678. - PubMed
-
- Schurr JM. The one-dimensional diffusion coefficient of proteins absorbed on DNA. Hydrodynamic considerations. Biophys. Chem. 1979;9:413–414. - PubMed
Publication types
MeSH terms
Substances
Grants and funding
LinkOut - more resources
Full Text Sources
Other Literature Sources