Modification of PATase by L/F-transferase generates a ClpS-dependent N-end rule substrate in Escherichia coli
- PMID: 19440203
- PMCID: PMC2699360
- DOI: 10.1038/emboj.2009.134
Modification of PATase by L/F-transferase generates a ClpS-dependent N-end rule substrate in Escherichia coli
Abstract
The N-end rule pathway is conserved from bacteria to man and determines the half-life of a protein based on its N-terminal amino acid. In Escherichia coli, model substrates bearing an N-degron are recognised by ClpS and degraded by ClpAP in an ATP-dependent manner. Here, we report the isolation of 23 ClpS-interacting proteins from E. coli. Our data show that at least one of these interacting proteins--putrescine aminotransferase (PATase)--is post-translationally modified to generate a primary N-degron. Remarkably, the N-terminal modification of PATase is generated by a new specificity of leucyl/phenylalanyl-tRNA-protein transferase (LFTR), in which various combinations of primary destabilising residues (Leu and Phe) are attached to the N-terminal Met. This modification (of PATase), by LFTR, is essential not only for its recognition by ClpS, but also determines the stability of the protein in vivo. Thus, the N-end rule pathway, through the ClpAPS-mediated turnover of PATase may have an important function in putrescine homeostasis. In addition, we have identified a new element within the N-degron, which is required for substrate delivery to ClpA.
Figures
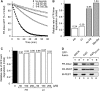
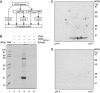
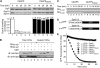
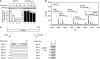
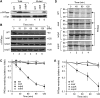
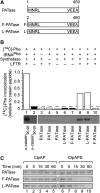
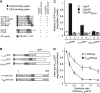
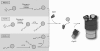
Similar articles
-
ClpS is the recognition component for Escherichia coli substrates of the N-end rule degradation pathway.Mol Microbiol. 2009 Apr;72(2):506-17. doi: 10.1111/j.1365-2958.2009.06666.x. Epub 2009 Mar 17. Mol Microbiol. 2009. PMID: 19317833
-
An intrinsic degradation tag on the ClpA C-terminus regulates the balance of ClpAP complexes with different substrate specificity.J Mol Biol. 2008 Dec 12;384(2):503-11. doi: 10.1016/j.jmb.2008.09.046. Epub 2008 Sep 26. J Mol Biol. 2008. PMID: 18835567
-
Structural basis of N-end rule substrate recognition in Escherichia coli by the ClpAP adaptor protein ClpS.EMBO Rep. 2009 May;10(5):508-14. doi: 10.1038/embor.2009.62. Epub 2009 Apr 17. EMBO Rep. 2009. PMID: 19373253 Free PMC article.
-
The molecular basis for the post-translational addition of amino acids by L/F transferase in the N-end rule pathway.Curr Protein Pept Sci. 2015;16(2):163-80. Curr Protein Pept Sci. 2015. PMID: 25692952 Review.
-
The N-end rule pathway for regulated proteolysis: prokaryotic and eukaryotic strategies.Trends Cell Biol. 2007 Apr;17(4):165-72. doi: 10.1016/j.tcb.2007.02.001. Epub 2007 Feb 15. Trends Cell Biol. 2007. PMID: 17306546 Review.
Cited by
-
ClpS1 is a conserved substrate selector for the chloroplast Clp protease system in Arabidopsis.Plant Cell. 2013 Jun;25(6):2276-301. doi: 10.1105/tpc.113.112557. Epub 2013 Jun 28. Plant Cell. 2013. PMID: 23898032 Free PMC article.
-
A single ClpS monomer is sufficient to direct the activity of the ClpA hexamer.J Biol Chem. 2010 Mar 19;285(12):8771-81. doi: 10.1074/jbc.M109.053736. Epub 2010 Jan 12. J Biol Chem. 2010. PMID: 20068042 Free PMC article.
-
Adapting the machine: adaptor proteins for Hsp100/Clp and AAA+ proteases.Nat Rev Microbiol. 2009 Aug;7(8):589-99. doi: 10.1038/nrmicro2185. Nat Rev Microbiol. 2009. PMID: 19609260 Review.
-
N-Terminal-Based Targeted, Inducible Protein Degradation in Escherichia coli.PLoS One. 2016 Feb 22;11(2):e0149746. doi: 10.1371/journal.pone.0149746. eCollection 2016. PLoS One. 2016. PMID: 26900850 Free PMC article.
-
Molecular and structural insights into an asymmetric proteolytic complex (ClpP1P2) from Mycobacterium smegmatis.Sci Rep. 2019 Dec 2;9(1):18019. doi: 10.1038/s41598-019-53736-8. Sci Rep. 2019. PMID: 31792243 Free PMC article.
References
-
- Almiron M, Link AJ, Furlong D, Kolter R (1992) A novel DNA-binding protein with regulatory and protective roles in starved Escherichia coli. Genes Dev 6: 2646–2654 - PubMed
Publication types
MeSH terms
Substances
LinkOut - more resources
Full Text Sources
Molecular Biology Databases
Research Materials
Miscellaneous