Postnatal differentiation of basket cells from slow to fast signaling devices
- PMID: 19036989
- PMCID: PMC6671784
- DOI: 10.1523/JNEUROSCI.2890-08.2008
Postnatal differentiation of basket cells from slow to fast signaling devices
Abstract
Gamma frequency (30-100 Hz) oscillations in the mature cortex underlie higher cognitive functions. Fast signaling in GABAergic interneuron networks plays a key role in the generation of these oscillations. During development of the rodent brain, gamma activity appears at the end of the first postnatal week, but frequency and synchrony reach adult levels only by the fourth week. However, the mechanisms underlying the maturation of gamma activity are unclear. Here we demonstrate that hippocampal basket cells (BCs), the proposed cellular substrate of gamma oscillations, undergo marked changes in their morphological, intrinsic, and synaptic properties between postnatal day 6 (P6) and P25. During maturation, action potential duration, propagation time, duration of the release period, and decay time constant of IPSCs decreases by approximately 30-60%. Thus, postnatal development converts BCs from slow into fast signaling devices. Computational analysis reveals that BC networks with young intrinsic and synaptic properties as well as reduced connectivity generate oscillations with moderate coherence in the lower gamma frequency range. In contrast, BC networks with mature properties and increased connectivity generate highly coherent activity in the upper gamma frequency band. Thus, late postnatal maturation of BCs enhances coherence in neuronal networks and will thereby contribute to the development of cognitive brain functions.
Figures
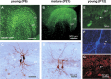
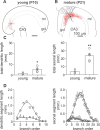
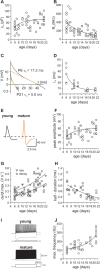
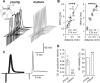
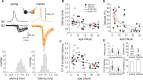
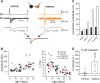
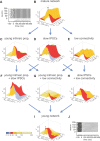
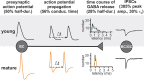
Similar articles
-
Signaling properties of stratum oriens interneurons in the hippocampus of transgenic mice expressing EGFP in a subset of somatostatin-containing cells.Hippocampus. 2007;17(7):538-53. doi: 10.1002/hipo.20291. Hippocampus. 2007. PMID: 17455332
-
Kainate receptors and signal integration by NG2 glial cells.Neuron Glia Biol. 2009 May;5(1-2):13-20. doi: 10.1017/S1740925X09990081. Epub 2009 Dec 22. Neuron Glia Biol. 2009. PMID: 20025816
-
Rapid signaling at inhibitory synapses in a dentate gyrus interneuron network.J Neurosci. 2001 Apr 15;21(8):2687-98. doi: 10.1523/JNEUROSCI.21-08-02687.2001. J Neurosci. 2001. PMID: 11306622 Free PMC article.
-
Highly energized inhibitory interneurons are a central element for information processing in cortical networks.J Cereb Blood Flow Metab. 2014 Aug;34(8):1270-82. doi: 10.1038/jcbfm.2014.104. Epub 2014 Jun 4. J Cereb Blood Flow Metab. 2014. PMID: 24896567 Free PMC article. Review.
-
Cellular mechanisms of neuronal population oscillations in the hippocampus in vitro.Annu Rev Neurosci. 2004;27:247-78. doi: 10.1146/annurev.neuro.27.070203.144303. Annu Rev Neurosci. 2004. PMID: 15217333 Review.
Cited by
-
SCN1A mutations in Dravet syndrome: impact of interneuron dysfunction on neural networks and cognitive outcome.Epilepsy Behav. 2012 Mar;23(3):177-86. doi: 10.1016/j.yebeh.2011.11.022. Epub 2012 Feb 16. Epilepsy Behav. 2012. PMID: 22341965 Free PMC article. Review.
-
Step by step: cells with multiple functions in cortical circuit assembly.Nat Rev Neurosci. 2022 Jul;23(7):395-410. doi: 10.1038/s41583-022-00585-6. Epub 2022 Apr 14. Nat Rev Neurosci. 2022. PMID: 35422526 Review.
-
Neuroligin-2 controls the establishment of fast GABAergic transmission in adult-born granule cells.Hippocampus. 2023 Apr;33(4):424-441. doi: 10.1002/hipo.23505. Epub 2023 Jan 28. Hippocampus. 2023. PMID: 36709408 Free PMC article.
-
Interactions between Membrane Resistance, GABA-A Receptor Properties, Bicarbonate Dynamics and Cl--Transport Shape Activity-Dependent Changes of Intracellular Cl- Concentration.Int J Mol Sci. 2019 Mar 20;20(6):1416. doi: 10.3390/ijms20061416. Int J Mol Sci. 2019. PMID: 30897846 Free PMC article.
-
Long-term sensory deprivation selectively rearranges functional inhibitory circuits in mouse barrel cortex.Proc Natl Acad Sci U S A. 2009 Jul 21;106(29):12156-61. doi: 10.1073/pnas.0900922106. Epub 2009 Jul 7. Proc Natl Acad Sci U S A. 2009. PMID: 19584253 Free PMC article.
References
-
- Bartos M, Vida I, Jonas P. Synaptic mechanisms of synchronized gamma oscillations in inhibitory interneuron networks. Nat Rev Neurosci. 2007;8:45–56. - PubMed
Publication types
MeSH terms
Substances
LinkOut - more resources
Full Text Sources
Other Literature Sources
Research Materials
Miscellaneous