Metabolic and hemodynamic events after changes in neuronal activity: current hypotheses, theoretical predictions and in vivo NMR experimental findings
- PMID: 19002199
- PMCID: PMC2743443
- DOI: 10.1038/jcbfm.2008.134
Metabolic and hemodynamic events after changes in neuronal activity: current hypotheses, theoretical predictions and in vivo NMR experimental findings
Abstract
Unraveling the energy metabolism and the hemodynamic outcomes of excitatory and inhibitory neuronal activity is critical not only for our basic understanding of overall brain function, but also for the understanding of many brain disorders. Methodologies of magnetic resonance spectroscopy (MRS) and magnetic resonance imaging (MRI) are powerful tools for the noninvasive investigation of brain metabolism and physiology. However, the temporal and spatial resolution of in vivo MRS and MRI is not suitable to provide direct evidence for hypotheses that involve metabolic compartmentalization between different cell types, or to untangle the complex neuronal microcircuitry, which results in changes of electrical activity. This review aims at describing how the current models of brain metabolism, mainly built on the basis of in vitro evidence, relate to experimental findings recently obtained in vivo by (1)H MRS, (13)C MRS, and MRI. The hypotheses related to the role of different metabolic substrates, the metabolic neuron-glia interactions, along with the available theoretical predictions of the energy budget of neurotransmission will be discussed. In addition, the cellular and network mechanisms that characterize different types of increased and suppressed neuronal activity will be considered within the sensitivity-constraints of MRS and MRI.
Figures
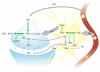
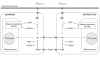
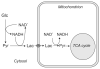
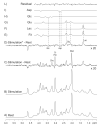
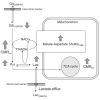
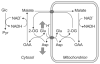
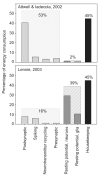
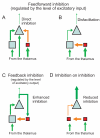
Similar articles
-
Biophysical basis of brain activity: implications for neuroimaging.Q Rev Biophys. 2002 Aug;35(3):287-325. doi: 10.1017/s0033583502003803. Q Rev Biophys. 2002. PMID: 12599751 Review.
-
Cellular mechanisms of brain energy metabolism and their relevance to functional brain imaging.Philos Trans R Soc Lond B Biol Sci. 1999 Jul 29;354(1387):1155-63. doi: 10.1098/rstb.1999.0471. Philos Trans R Soc Lond B Biol Sci. 1999. PMID: 10466143 Free PMC article. Review.
-
In vivo 1H-[13C]-NMR spectroscopy of cerebral metabolism.NMR Biomed. 2003 Oct-Nov;16(6-7):339-57. doi: 10.1002/nbm.847. NMR Biomed. 2003. PMID: 14679499 Review.
-
In vivo NMR studies of the glutamate neurotransmitter flux and neuroenergetics: implications for brain function.Annu Rev Physiol. 2003;65:401-27. doi: 10.1146/annurev.physiol.65.092101.142131. Epub 2002 May 1. Annu Rev Physiol. 2003. PMID: 12524459 Review.
-
Modelling of the coupling between brain electrical activity and metabolism.Acta Biotheor. 2001;49(4):301-26. doi: 10.1023/a:1014286728421. Acta Biotheor. 2001. PMID: 11804241
Cited by
-
Influence of Repetitive Transcranial Magnetic Stimulation on Human Neurochemistry and Functional Connectivity: A Pilot MRI/MRS Study at 7 T.Front Neurosci. 2019 Nov 27;13:1260. doi: 10.3389/fnins.2019.01260. eCollection 2019. Front Neurosci. 2019. PMID: 31827419 Free PMC article.
-
Lactate transport and metabolism in the human brain: implications for the astrocyte-neuron lactate shuttle hypothesis.J Neurosci. 2011 Mar 30;31(13):4768-70. doi: 10.1523/JNEUROSCI.6612-10.2011. J Neurosci. 2011. PMID: 21451014 Free PMC article. No abstract available.
-
Learning-Induced Gene Expression in the Hippocampus Reveals a Role of Neuron -Astrocyte Metabolic Coupling in Long Term Memory.PLoS One. 2015 Oct 29;10(10):e0141568. doi: 10.1371/journal.pone.0141568. eCollection 2015. PLoS One. 2015. PMID: 26513352 Free PMC article.
-
Neurochemical and BOLD responses during neuronal activation measured in the human visual cortex at 7 Tesla.J Cereb Blood Flow Metab. 2015 Mar 31;35(4):601-10. doi: 10.1038/jcbfm.2014.233. J Cereb Blood Flow Metab. 2015. PMID: 25564236 Free PMC article.
-
Windows on the human body--in vivo high-field magnetic resonance research and applications in medicine and psychology.Sensors (Basel). 2010;10(6):5724-57. doi: 10.3390/s100605724. Epub 2010 Jun 8. Sensors (Basel). 2010. PMID: 22219684 Free PMC article.
References
-
- Ames A., 3rd CNS energy metabolism as related to function. Brain Res Brain Res Rev. 2000;34:42–68. - PubMed
-
- Amit DJ, Brunel N. Model of global spontaneous activity and local structured activity during delay periods in the cerebral cortex. Cereb Cortex. 1997;7:237–52. - PubMed
-
- Atlante A, de Bari L, Bobba A, Marra E, Passarella S. Transport and metabolism of L-lactate occur in mitochondria from cerebellar granule cells and are modified in cells undergoing low potassium dependent apoptosis. Biochim Biophys Acta. 2007;1767:1285–99. - PubMed
-
- Attwell D, Iadecola C. The neural basis of functional brain imaging signals. Trends Neurosci. 2002;25:621–5. - PubMed
Publication types
MeSH terms
Substances
Grants and funding
LinkOut - more resources
Full Text Sources