Myosin Vb mobilizes recycling endosomes and AMPA receptors for postsynaptic plasticity
- PMID: 18984164
- PMCID: PMC2585749
- DOI: 10.1016/j.cell.2008.09.057
Myosin Vb mobilizes recycling endosomes and AMPA receptors for postsynaptic plasticity
Abstract
Learning-related plasticity at excitatory synapses in the mammalian brain requires the trafficking of AMPA receptors and the growth of dendritic spines. However, the mechanisms that couple plasticity stimuli to the trafficking of postsynaptic cargo are poorly understood. Here we demonstrate that myosin Vb (MyoVb), a Ca2+-sensitive motor, conducts spine trafficking during long-term potentiation (LTP) of synaptic strength. Upon activation of NMDA receptors and corresponding Ca2+ influx, MyoVb associates with recycling endosomes (REs), triggering rapid spine recruitment of endosomes and local exocytosis in spines. Disruption of MyoVb or its interaction with the RE adaptor Rab11-FIP2 abolishes LTP-induced exocytosis from REs and prevents both AMPA receptor insertion and spine growth. Furthermore, induction of tight binding of MyoVb to actin using an acute chemical genetic strategy eradicates LTP in hippocampal slices. Thus, Ca2+-activated MyoVb captures and mobilizes REs for AMPA receptor insertion and spine growth, providing a mechanistic link between the induction and expression of postsynaptic plasticity.
Figures
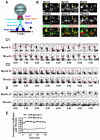
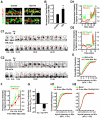
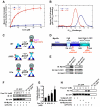
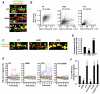
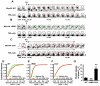
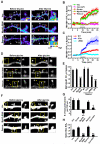
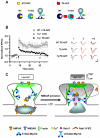
Comment in
-
Myosin learns to recruit AMPA receptors.Cell. 2008 Oct 31;135(3):414-5. doi: 10.1016/j.cell.2008.10.013. Cell. 2008. PMID: 18984153
Similar articles
-
Recycling endosomes supply AMPA receptors for LTP.Science. 2004 Sep 24;305(5692):1972-5. doi: 10.1126/science.1102026. Science. 2004. PMID: 15448273
-
A retention-release mechanism based on RAB11FIP2 for AMPA receptor synaptic delivery during long-term potentiation.J Cell Sci. 2019 Dec 16;132(24):jcs234237. doi: 10.1242/jcs.234237. J Cell Sci. 2019. PMID: 31757887
-
New insights in endosomal dynamics and AMPA receptor trafficking.Semin Cell Dev Biol. 2011 Jul;22(5):499-505. doi: 10.1016/j.semcdb.2011.06.008. Epub 2011 Aug 6. Semin Cell Dev Biol. 2011. PMID: 21843653 Review.
-
Motor protein-dependent transport of AMPA receptors into spines during long-term potentiation.Nat Neurosci. 2008 Apr;11(4):457-66. doi: 10.1038/nn2063. Epub 2008 Mar 2. Nat Neurosci. 2008. PMID: 18311135
-
Role of syntaxin 4 in activity-dependent exocytosis and synaptic plasticity in hippocampal neurons.Sci Signal. 2010 Oct 19;3(144):jc7. doi: 10.1126/scisignal.3144jc7. Sci Signal. 2010. PMID: 20959521 Review.
Cited by
-
The functional interplay of Rab11, FIP3 and Rho proteins on the endosomal recycling pathway controls cell shape and symmetry.Small GTPases. 2018 Jul 4;9(4):310-315. doi: 10.1080/21541248.2016.1224288. Epub 2016 Sep 2. Small GTPases. 2018. PMID: 27533792 Free PMC article. Review.
-
Imaging pHluorin-tagged receptor insertion to the plasma membrane in primary cultured mouse neurons.J Vis Exp. 2012 Nov 20;(69):4450. doi: 10.3791/4450. J Vis Exp. 2012. PMID: 23208071 Free PMC article.
-
The dendritic SNARE fusion machinery involved in AMPARs insertion during long-term potentiation.Front Cell Neurosci. 2014 Dec 22;8:407. doi: 10.3389/fncel.2014.00407. eCollection 2014. Front Cell Neurosci. 2014. PMID: 25565955 Free PMC article. Review.
-
Naltrexone Facilitates Learning and Delays Extinction by Increasing AMPA Receptor Phosphorylation and Membrane Insertion.Biol Psychiatry. 2016 Jun 1;79(11):906-16. doi: 10.1016/j.biopsych.2015.04.019. Epub 2015 May 2. Biol Psychiatry. 2016. PMID: 26049209 Free PMC article.
-
Regulation of NMDA receptor transport: a KIF17-cargo binding/releasing underlies synaptic plasticity and memory in vivo.J Neurosci. 2012 Apr 18;32(16):5486-99. doi: 10.1523/JNEUROSCI.0718-12.2012. J Neurosci. 2012. PMID: 22514311 Free PMC article.
References
-
- Alvarez VA, Sabatini BL. Anatomical and physiological plasticity of dendritic spines. Annual review of neuroscience. 2007;30:79–97. - PubMed
-
- Blanpied TA, Scott DB, Ehlers MD. Dynamics and regulation of clathrin coats at specialized endocytic zones of dendrites and spines. Neuron. 2002;36:435–449. - PubMed
-
- Correia SS, Bassani S, Brown TC, Lise MF, Backos DS, El-Husseini A, Passafaro M, Esteban JA. Motor protein-dependent transport of AMPA receptors into spines during long-term potentiation. Nature neuroscience. 2008;11:457–466. - PubMed
-
- Derkach VA, Oh MC, Guire ES, Soderling TR. Regulatory mechanisms of AMPA receptors in synaptic plasticity. Nature reviews. 2007;8:101–113. - PubMed
Publication types
MeSH terms
Substances
Grants and funding
LinkOut - more resources
Full Text Sources
Other Literature Sources
Molecular Biology Databases
Miscellaneous