Highly selective receptive fields in mouse visual cortex
- PMID: 18650330
- PMCID: PMC3040721
- DOI: 10.1523/JNEUROSCI.0623-08.2008
Highly selective receptive fields in mouse visual cortex
Abstract
Genetic methods available in mice are likely to be powerful tools in dissecting cortical circuits. However, the visual cortex, in which sensory coding has been most thoroughly studied in other species, has essentially been neglected in mice perhaps because of their poor spatial acuity and the lack of columnar organization such as orientation maps. We have now applied quantitative methods to characterize visual receptive fields in mouse primary visual cortex V1 by making extracellular recordings with silicon electrode arrays in anesthetized mice. We used current source density analysis to determine laminar location and spike waveforms to discriminate putative excitatory and inhibitory units. We find that, although the spatial scale of mouse receptive fields is up to one or two orders of magnitude larger, neurons show selectivity for stimulus parameters such as orientation and spatial frequency that is near to that found in other species. Furthermore, typical response properties such as linear versus nonlinear spatial summation (i.e., simple and complex cells) and contrast-invariant tuning are also present in mouse V1 and correlate with laminar position and cell type. Interestingly, we find that putative inhibitory neurons generally have less selective, and nonlinear, responses. This quantitative description of receptive field properties should facilitate the use of mouse visual cortex as a system to address longstanding questions of visual neuroscience and cortical processing.
Figures
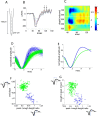
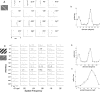
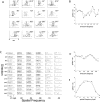
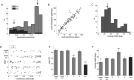
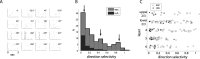
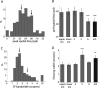
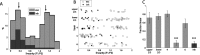
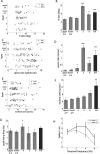
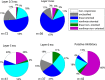
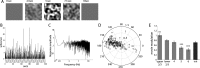
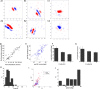
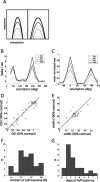
Similar articles
-
Nonlinear Y-Like Receptive Fields in the Early Visual Cortex: An Intermediate Stage for Building Cue-Invariant Receptive Fields from Subcortical Y Cells.J Neurosci. 2017 Jan 25;37(4):998-1013. doi: 10.1523/JNEUROSCI.2120-16.2016. J Neurosci. 2017. PMID: 28123031 Free PMC article.
-
Comparison of visual receptive field properties of the superior colliculus and primary visual cortex in rats.Brain Res Bull. 2015 Aug;117:69-80. doi: 10.1016/j.brainresbull.2015.07.007. Epub 2015 Jul 26. Brain Res Bull. 2015. PMID: 26222378
-
Orientation selectivity of synaptic input to neurons in mouse and cat primary visual cortex.J Neurosci. 2011 Aug 24;31(34):12339-50. doi: 10.1523/JNEUROSCI.2039-11.2011. J Neurosci. 2011. PMID: 21865476 Free PMC article.
-
The contribution of vertical and horizontal connections to the receptive field center and surround in V1.Neural Netw. 2004 Jun-Jul;17(5-6):681-93. doi: 10.1016/j.neunet.2004.05.002. Neural Netw. 2004. PMID: 15288892 Review.
-
Contribution of feedforward, lateral and feedback connections to the classical receptive field center and extra-classical receptive field surround of primate V1 neurons.Prog Brain Res. 2006;154:93-120. doi: 10.1016/S0079-6123(06)54005-1. Prog Brain Res. 2006. PMID: 17010705 Review.
Cited by
-
Impact of Perineuronal Nets on Electrophysiology of Parvalbumin Interneurons, Principal Neurons, and Brain Oscillations: A Review.Front Synaptic Neurosci. 2021 May 10;13:673210. doi: 10.3389/fnsyn.2021.673210. eCollection 2021. Front Synaptic Neurosci. 2021. PMID: 34040511 Free PMC article.
-
Tug-of-Peace: Visual Rivalry and Atypical Visual Motion Processing in MECP2 Duplication Syndrome of Autism.eNeuro. 2024 Jan 16;11(1):ENEURO.0102-23.2023. doi: 10.1523/ENEURO.0102-23.2023. Print 2024 Jan. eNeuro. 2024. PMID: 37940561 Free PMC article.
-
Cortical Feedback Regulates Feedforward Retinogeniculate Refinement.Neuron. 2016 Sep 7;91(5):1021-1033. doi: 10.1016/j.neuron.2016.07.040. Epub 2016 Aug 18. Neuron. 2016. PMID: 27545712 Free PMC article.
-
Dendritic BDNF synthesis is required for late-phase spine maturation and recovery of cortical responses following sensory deprivation.J Neurosci. 2012 Apr 4;32(14):4790-802. doi: 10.1523/JNEUROSCI.4462-11.2012. J Neurosci. 2012. PMID: 22492034 Free PMC article.
-
Improving data quality in neuronal population recordings.Nat Neurosci. 2016 Aug 26;19(9):1165-74. doi: 10.1038/nn.4365. Nat Neurosci. 2016. PMID: 27571195 Free PMC article. Review.
References
-
- Andermann ML, Ritt J, Neimark MA, Moore CI. Neural correlates of vibrissa resonance; band-pass and somatotopic representation of high-frequency stimuli. Neuron. 2004;42:451–463. - PubMed
-
- Anderson JS, Carandini M, Ferster D. Orientation tuning of input conductance, excitation, and inhibition in cat primary visual cortex. J Neurophysiol. 2000;84:909–926. - PubMed
-
- Barthó P, Hirase H, Monconduit L, Zugaro M, Harris KD, Buzsáki G. Characterization of neocortical principal cells and interneurons by network interactions and extracellular features. J Neurophysiol. 2004;92:600–608. - PubMed
Publication types
MeSH terms
Grants and funding
LinkOut - more resources
Full Text Sources
Other Literature Sources