Assessment of tissue redox status using metabolic responsive contrast agents and magnetic resonance imaging
- PMID: 18644197
- PMCID: PMC2752670
- DOI: 10.1211/jpp.60.8.0011
Assessment of tissue redox status using metabolic responsive contrast agents and magnetic resonance imaging
Abstract
Regulation of tissue redox status is important to maintain normal physiological conditions in the living body. Disruption of redox homoeostasis may lead to oxidative stress and can induce many pathological conditions such as cancer, neurological disorders and ageing. Therefore, imaging of tissue redox status could have clinical applications. Redox imaging employing magnetic resonance imaging (MRI) with nitroxides as cell-permeable redox-sensitive contrast agents has been used for non-invasive monitoring of tissue redox status in animal models. The redox imaging applications of nitroxide electron paramagnetic resonance imaging (EPRI) and MRI are reviewed here, with a focus on application of tumour redox status monitoring. While particular emphasis has been placed on differences in the redox status in tumours compared to selected normal tissues, the technique possesses the potential to have broad applications to the study of other disease states, inflammatory processes and other circumstances where oxidative stress is implicated.
Figures
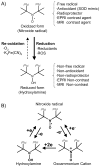
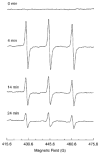
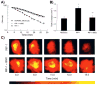
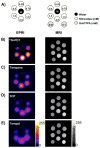
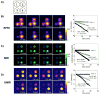
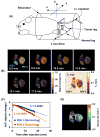
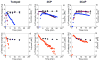
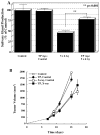
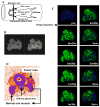
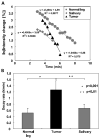
Similar articles
-
[Redox Molecular Imaging Using ReMI].Yakugaku Zasshi. 2015;135(5):725-31. doi: 10.1248/yakushi.14-00235-2. Yakugaku Zasshi. 2015. PMID: 25948309 Review. Japanese.
-
Brain imaging in methamphetamine-treated mice using a nitroxide contrast agent for EPR imaging of the redox status and a gadolinium contrast agent for MRI observation of blood-brain barrier function.Free Radic Res. 2015;49(8):1038-47. doi: 10.3109/10715762.2015.1040787. Epub 2015 Jul 3. Free Radic Res. 2015. PMID: 25968953
-
In vivo evaluation of different alterations of redox status by studying pharmacokinetics of nitroxides using magnetic resonance techniques.Redox Biol. 2016 Aug;8:226-42. doi: 10.1016/j.redox.2015.10.007. Epub 2015 Nov 14. Redox Biol. 2016. PMID: 26827126 Free PMC article. Review.
-
Monitoring redox-sensitive paramagnetic contrast agent by EPRI, OMRI and MRI.J Magn Reson. 2008 Jan;190(1):105-12. doi: 10.1016/j.jmr.2007.10.013. Epub 2007 Oct 30. J Magn Reson. 2008. PMID: 18006345 Free PMC article.
-
High-resolution mapping of tumor redox status by magnetic resonance imaging using nitroxides as redox-sensitive contrast agents.Clin Cancer Res. 2006 Apr 15;12(8):2455-62. doi: 10.1158/1078-0432.CCR-05-2747. Clin Cancer Res. 2006. PMID: 16638852
Cited by
-
Oxidative stress evaluation of skeletal muscle in ischemia-reperfusion injury using enhanced magnetic resonance imaging.Sci Rep. 2020 Jul 2;10(1):10863. doi: 10.1038/s41598-020-67336-4. Sci Rep. 2020. PMID: 32616815 Free PMC article.
-
Alternatives to gadolinium-based metal chelates for magnetic resonance imaging.Chem Rev. 2010 May 12;110(5):2960-3018. doi: 10.1021/cr900284a. Chem Rev. 2010. PMID: 20397688 Free PMC article. Review. No abstract available.
-
Collective activation of MRI agents via encapsulation and disease-triggered release.J Am Chem Soc. 2013 May 29;135(21):7847-50. doi: 10.1021/ja403167p. Epub 2013 May 17. J Am Chem Soc. 2013. PMID: 23672342 Free PMC article.
-
Effects of tempol and redox-cycling nitroxides in models of oxidative stress.Pharmacol Ther. 2010 May;126(2):119-45. doi: 10.1016/j.pharmthera.2010.01.003. Epub 2010 Feb 11. Pharmacol Ther. 2010. PMID: 20153367 Free PMC article. Review.
-
Noninvasive Diagnosis of the Mitochondrial Function of Doxorubicin-Induced Cardiomyopathy Using In Vivo Dynamic Nuclear Polarization-Magnetic Resonance Imaging.Antioxidants (Basel). 2022 Jul 26;11(8):1454. doi: 10.3390/antiox11081454. Antioxidants (Basel). 2022. PMID: 35892655 Free PMC article.
References
-
- Barbier EL, Lamalle L, Decorps M. Methodology of brain perfusion imaging. J Magn Reson Imaging. 2001;13:496–520. - PubMed
-
- Berliner JL, Fujii H. Magnetic resonance imaging of biological specimens by electron paramagnetic resonance of nitroxide spin labels. Science. 1985;227:517–9. - PubMed
-
- Berliner LJ, Fujii H, Wan XM, Lukiewicz SJ. Feasibility study of imaging a living murine tumor by electron paramagnetic resonance. Magn Reson Med. 1987;4:380–4. - PubMed
-
- Brasch RC. Work in progress: methods of contrast enhancement for NMR imaging and potential applications. A subject review. Radiology. 1983;147:781–8. - PubMed
-
- Brasch RC, London DA, Wesbey GE, Tozer TN, Nitecki DE, Williams RD, Doemeny J, Tuck LD, Lallemand DP. Work in progress: nuclear magnetic resonance study of a paramagnetic nitroxide contrast agent for enhancement of renal structures in experimental animals. Radiology. 1983;147:773–9. - PubMed
Publication types
MeSH terms
Substances
Grants and funding
LinkOut - more resources
Full Text Sources
Other Literature Sources
Medical