How force might activate talin's vinculin binding sites: SMD reveals a structural mechanism
- PMID: 18282082
- PMCID: PMC2242828
- DOI: 10.1371/journal.pcbi.0040024
How force might activate talin's vinculin binding sites: SMD reveals a structural mechanism
Abstract
Upon cell adhesion, talin physically couples the cytoskeleton via integrins to the extracellular matrix, and subsequent vinculin recruitment is enhanced by locally applied tensile force. Since the vinculin binding (VB) sites are buried in the talin rod under equilibrium conditions, the structural mechanism of how vinculin binding to talin is force-activated remains unknown. Taken together with experimental data, a biphasic vinculin binding model, as derived from steered molecular dynamics, provides high resolution structural insights how tensile mechanical force applied to the talin rod fragment (residues 486-889 constituting helices H1-H12) might activate the VB sites. Fragmentation of the rod into three helix subbundles is prerequisite to the sequential exposure of VB helices to water. Finally, unfolding of a VB helix into a completely stretched polypeptide might inhibit further binding of vinculin. The first events in fracturing the H1-H12 rods of talin1 and talin2 in subbundles are similar. The proposed force-activated alpha-helix swapping mechanism by which vinculin binding sites in talin rods are exposed works distinctly different from that of other force-activated bonds, including catch bonds.
Conflict of interest statement
Figures
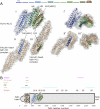
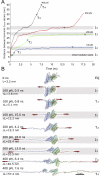
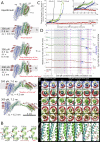
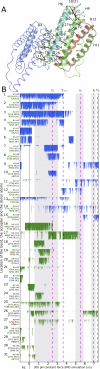
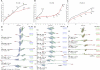
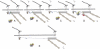
Similar articles
-
Molecular dynamics study of talin-vinculin binding.Biophys J. 2008 Aug;95(4):2027-36. doi: 10.1529/biophysj.107.124487. Epub 2008 Apr 11. Biophys J. 2008. PMID: 18408041 Free PMC article.
-
Stretching single talin rod molecules activates vinculin binding.Science. 2009 Jan 30;323(5914):638-41. doi: 10.1126/science.1162912. Science. 2009. PMID: 19179532 Free PMC article.
-
Molecular dynamics simulations reveal how vinculin refolds partially unfolded talin rod helices to stabilize them against mechanical force.PLoS Comput Biol. 2024 Aug 7;20(8):e1012341. doi: 10.1371/journal.pcbi.1012341. eCollection 2024 Aug. PLoS Comput Biol. 2024. PMID: 39110765 Free PMC article.
-
Integrin connections to the cytoskeleton through talin and vinculin.Biochem Soc Trans. 2008 Apr;36(Pt 2):235-9. doi: 10.1042/BST0360235. Biochem Soc Trans. 2008. PMID: 18363566 Review.
-
Cytoskeletal proteins talin and vinculin in integrin-mediated adhesion.Biochem Soc Trans. 2004 Nov;32(Pt 5):831-6. doi: 10.1042/BST0320831. Biochem Soc Trans. 2004. PMID: 15494027 Review.
Cited by
-
Arginylation-dependent regulation of a proteolytic product of talin is essential for cell-cell adhesion.J Cell Biol. 2012 Jun 11;197(6):819-36. doi: 10.1083/jcb.201112129. Epub 2012 Jun 4. J Cell Biol. 2012. PMID: 22665520 Free PMC article.
-
Cell fate regulation by coupling mechanical cycles to biochemical signaling pathways.Curr Opin Cell Biol. 2009 Feb;21(1):38-46. doi: 10.1016/j.ceb.2009.01.002. Epub 2009 Feb 11. Curr Opin Cell Biol. 2009. PMID: 19217273 Free PMC article. Review.
-
Molecular and structural insight into the role of key residues of thrombospondin-1 and calreticulin in thrombospondin-1-calreticulin binding.Biochemistry. 2011 Feb 1;50(4):566-73. doi: 10.1021/bi101639y. Epub 2011 Jan 4. Biochemistry. 2011. PMID: 21142150 Free PMC article.
-
An efficient alpha helix model and simulation framework for stationary electrostatic interaction force estimation.Sci Rep. 2021 Apr 27;11(1):9053. doi: 10.1038/s41598-021-88369-3. Sci Rep. 2021. PMID: 33907198 Free PMC article.
-
The interaction of vinculin with actin.PLoS Comput Biol. 2013 Apr;9(4):e1002995. doi: 10.1371/journal.pcbi.1002995. Epub 2013 Apr 25. PLoS Comput Biol. 2013. PMID: 23633939 Free PMC article.
References
-
- Jiang G, Giannone G, Critchley DR, Fukumoto E, Sheetz MP. Two-piconewton slip bond between fibronectin and the cytoskeleton depends on talin. Nature. 2003;424:334–337. - PubMed
-
- Calderwood DA, Ginsberg MH. Talin forges the links between integrins and actin. Nat Cell Biol. 2003;5:694–697. - PubMed
-
- Calderwood DA, Zent R, Grant R, Rees DJ, Hynes RO, et al. The talin head domain binds to integrin beta subunit cytoplasmic tails and regulates integrin activation. J Biol Chem. 1999;274:28071–28074. - PubMed
-
- Barsukov IL, Prescot A, Bate N, Patel B, Floyd DN, et al. Phosphatidylinositol phosphate kinase type 1gamma and beta1-integrin cytoplasmic domain bind to the same region in the talin FERM domain. J Biol Chem. 2003;278:31202–31209. - PubMed
Publication types
MeSH terms
Substances
LinkOut - more resources
Full Text Sources