In vivo simultaneous tracing and Ca(2+) imaging of local neuronal circuits
- PMID: 17359915
- PMCID: PMC1892750
- DOI: 10.1016/j.neuron.2007.02.018
In vivo simultaneous tracing and Ca(2+) imaging of local neuronal circuits
Abstract
A central question about the brain is how information is processed by large populations of neurons embedded in intricate local networks. Answering this question requires not only monitoring functional dynamics of many neurons simultaneously, but also interpreting such activity patterns in the context of neuronal circuitry. Here, we introduce a versatile approach for loading Ca(2+) indicators in vivo by local electroporation. With this method, Ca(2+) imaging can be performed both at neuron population level and with exquisite subcellular resolution down to dendritic spines and axon boutons. This enabled mitral cell odor-evoked ensemble activity to be analyzed simultaneously with revealing their specific connectivity to different glomeruli. Colabeling of Purkinje cell dendrites and intersecting parallel fibers allowed Ca(2+) imaging of both presynaptic boutons and postsynaptic dendrites. This approach thus provides an unprecedented capability for in vivo visualizing active cell ensembles and tracing their underlying local neuronal circuits.
Figures
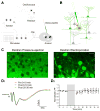
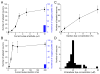
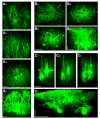
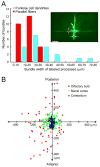
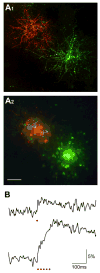
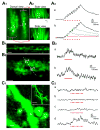
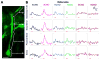
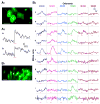
Comment in
-
Little strokes fill big oaks: a simple in vivo stain of brain cells.Neuron. 2007 Mar 15;53(6):771-3. doi: 10.1016/j.neuron.2007.03.001. Neuron. 2007. PMID: 17359911
Similar articles
-
In vivo calcium imaging of circuit activity in cerebellar cortex.J Neurophysiol. 2005 Aug;94(2):1636-44. doi: 10.1152/jn.01013.2004. Epub 2005 Apr 20. J Neurophysiol. 2005. PMID: 16079125
-
In vivo local dye electroporation for Ca²⁺ imaging and neuronal-circuit tracing.Cold Spring Harb Protoc. 2014 Sep 2;2014(9):940-7. doi: 10.1101/pdb.prot083501. Cold Spring Harb Protoc. 2014. PMID: 25183821
-
Calcium indicator loading of neurons using single-cell electroporation.Pflugers Arch. 2007 Jul;454(4):675-88. doi: 10.1007/s00424-007-0234-2. Epub 2007 Mar 2. Pflugers Arch. 2007. PMID: 17334778
-
Dendritic release of retrograde messengers controls synaptic transmission in local neocortical networks.Neuroscientist. 2005 Aug;11(4):334-44. doi: 10.1177/1073858405275827. Neuroscientist. 2005. PMID: 16061520 Review.
-
In vivo calcium imaging to illuminate neurocircuit activity dynamics underlying naturalistic behavior.Neuropsychopharmacology. 2015 Jan;40(1):238-9. doi: 10.1038/npp.2014.206. Neuropsychopharmacology. 2015. PMID: 25482169 Free PMC article. Review. No abstract available.
Cited by
-
Architecture of a mammalian glomerular domain revealed by novel volume electroporation using nanoengineered microelectrodes.Nat Commun. 2018 Jan 12;9(1):183. doi: 10.1038/s41467-017-02560-7. Nat Commun. 2018. PMID: 29330458 Free PMC article.
-
Regulation and function of adult neurogenesis: from genes to cognition.Physiol Rev. 2014 Oct;94(4):991-1026. doi: 10.1152/physrev.00004.2014. Physiol Rev. 2014. PMID: 25287858 Free PMC article. Review.
-
A simple and fast method to image calcium activity of neurons from intact dorsal root ganglia using fluorescent chemical Ca2+ indicators.Mol Pain. 2017 Jan-Dec;13:1744806917748051. doi: 10.1177/1744806917748051. Epub 2017 Dec 6. Mol Pain. 2017. PMID: 29212403 Free PMC article.
-
Mitochondrial calcium homeostasis: Implications for neurovascular and neurometabolic coupling.J Cereb Blood Flow Metab. 2017 Feb;37(2):381-395. doi: 10.1177/0271678X16680637. Epub 2016 Nov 24. J Cereb Blood Flow Metab. 2017. PMID: 27879386 Free PMC article. Review.
-
SeeDB: a simple and morphology-preserving optical clearing agent for neuronal circuit reconstruction.Nat Neurosci. 2013 Aug;16(8):1154-61. doi: 10.1038/nn.3447. Epub 2013 Jun 23. Nat Neurosci. 2013. PMID: 23792946
References
-
- Beierlein M, Gee KR, Martin VV, Regehr WG. Presynaptic calcium measurements at physiological temperatures using a new class of dextran-conjugated indicators. J Neurophysiol. 2004;92:591–599. - PubMed
-
- Bonnot A, Mentis GZ, Skoch J, O’Donovan MJ. Electroporation loading of calcium-sensitive dyes into the CNS. J Neurophysiol. 2005;93:1793–1808. - PubMed
-
- Bozza T, McGann JP, Mombaerts P, Wachowiak M. In vivo imaging of neuronal activity by targeted expression of a genetically encoded probe in the mouse. Neuron. 2004;42:9–21. - PubMed
Publication types
MeSH terms
Substances
Grants and funding
LinkOut - more resources
Full Text Sources
Other Literature Sources
Medical
Miscellaneous