Synaptotagmin-2 is essential for survival and contributes to Ca2+ triggering of neurotransmitter release in central and neuromuscular synapses
- PMID: 17192432
- PMCID: PMC6674714
- DOI: 10.1523/JNEUROSCI.3519-06.2006
Synaptotagmin-2 is essential for survival and contributes to Ca2+ triggering of neurotransmitter release in central and neuromuscular synapses
Abstract
Biochemical and genetic data suggest that synaptotagmin-2 functions as a Ca2+ sensor for fast neurotransmitter release in caudal brain regions, but animals and/or synapses lacking synaptotagmin-2 have not been examined. We have now generated mice in which the 5' end of the synaptotagmin-2 gene was replaced by lacZ. Using beta-galactosidase as a marker, we show that, consistent with previous studies, synaptotagmin-2 is widely expressed in spinal cord, brainstem, and cerebellum, but is additionally present in selected forebrain neurons, including most striatal neurons and some hypothalamic, cortical, and hippocampal neurons. Synaptotagmin-2-deficient mice were indistinguishable from wild-type littermates at birth, but subsequently developed severe motor dysfunction, and perished at approximately 3 weeks of age. Electrophysiological studies in cultured striatal neurons revealed that the synaptotagmin-2 deletion slowed the kinetics of evoked neurotransmitter release without altering the total amount of release. In contrast, synaptotagmin-2-deficient neuromuscular junctions (NMJs) suffered from a large reduction in evoked release and changes in short-term synaptic plasticity. Furthermore, in mutant NMJs, the frequency of spontaneous miniature release events was increased both at rest and during stimulus trains. Viewed together, our results demonstrate that the synaptotagmin-2 deficiency causes a lethal impairment in synaptic transmission in selected synapses. This impairment, however, is less severe than that produced in forebrain neurons by deletion of synaptotagmin-1, presumably because at least in NMJs, synaptotagmin-1 is coexpressed with synaptotagmin-2, and both together mediate fast Ca2+-triggered release. Thus, synaptotagmin-2 is an essential synaptotagmin isoform that functions in concert with other synaptotagmins in the Ca2+ triggering of neurotransmitter release.
Figures
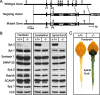
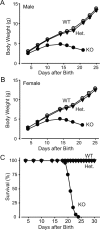
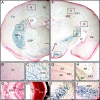
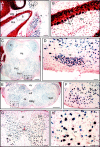
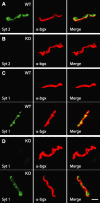
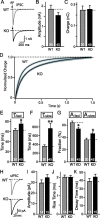
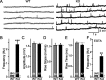
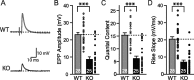
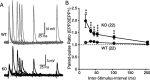
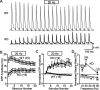
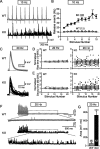
Comment in
-
Synaptotagmin: is 2 better than 1?J Neurosci. 2007 Apr 18;27(16):4231-2. doi: 10.1523/JNEUROSCI.0668-07.2007. J Neurosci. 2007. PMID: 17442806 Free PMC article. Review. No abstract available.
Similar articles
-
Genetic analysis of synaptotagmin 2 in spontaneous and Ca2+-triggered neurotransmitter release.EMBO J. 2006 May 17;25(10):2039-50. doi: 10.1038/sj.emboj.7601103. Epub 2006 Apr 27. EMBO J. 2006. PMID: 16642042 Free PMC article.
-
Synaptotagmin I: a major Ca2+ sensor for transmitter release at a central synapse.Cell. 1994 Nov 18;79(4):717-27. doi: 10.1016/0092-8674(94)90556-8. Cell. 1994. PMID: 7954835
-
Synaptotagmin2 (Syt2) Drives Fast Release Redundantly with Syt1 at the Output Synapses of Parvalbumin-Expressing Inhibitory Neurons.J Neurosci. 2017 Apr 26;37(17):4604-4617. doi: 10.1523/JNEUROSCI.3736-16.2017. Epub 2017 Mar 31. J Neurosci. 2017. PMID: 28363983 Free PMC article.
-
Calcium channels involved in neurotransmitter release at adult, neonatal and P/Q-type deficient neuromuscular junctions (Review).Mol Membr Biol. 2002 Oct-Dec;19(4):293-300. doi: 10.1080/0968768021000035087. Mol Membr Biol. 2002. PMID: 12512776 Review.
-
Synaptotagmin I, a Ca2+ sensor for neurotransmitter release.Trends Neurosci. 2003 Aug;26(8):413-22. doi: 10.1016/S0166-2236(03)00195-4. Trends Neurosci. 2003. PMID: 12900172 Review.
Cited by
-
Molecular underpinnings of synaptic vesicle pool heterogeneity.Traffic. 2015 Apr;16(4):338-64. doi: 10.1111/tra.12262. Traffic. 2015. PMID: 25620674 Free PMC article. Review.
-
Identification of a new SYT2 variant validates an unusual distal motor neuropathy phenotype.Neurol Genet. 2018 Oct 22;4(6):e282. doi: 10.1212/NXG.0000000000000282. eCollection 2018 Dec. Neurol Genet. 2018. PMID: 30533528 Free PMC article.
-
Inhibition of calcium-triggered secretion by hydrocarbon-stapled peptides.Nature. 2022 Mar;603(7903):949-956. doi: 10.1038/s41586-022-04543-1. Epub 2022 Mar 23. Nature. 2022. PMID: 35322233 Free PMC article.
-
Cholinergic efferent synaptic transmission regulates the maturation of auditory hair cell ribbon synapses.Open Biol. 2013 Nov;3(11):130163. doi: 10.1098/rsob.130163. Open Biol. 2013. PMID: 24350389 Free PMC article.
-
Neuronal calcium sensor synaptotagmin-9 is not involved in the regulation of glucose homeostasis or insulin secretion.PLoS One. 2010 Nov 9;5(11):e15414. doi: 10.1371/journal.pone.0015414. PLoS One. 2010. PMID: 21085706 Free PMC article.
References
-
- Buffelli M, Burgess RW, Feng G, Lobe CG, Lichtman JW, Sanes JR. Genetic evidence that relative synaptic efficacy biases the outcome of synaptic competition. Nature. 2003;424:430–434. - PubMed
-
- Cruz LJ, Gray WR, Olivera BM, Zeikus RD, Kerr L, Yoshikami D, Moczydlowski E. Conus geographus toxins that discriminate between neuronal and muscle sodium channels. J Biol Chem. 1985;260:9280–9288. - PubMed
Publication types
MeSH terms
Substances
Grants and funding
LinkOut - more resources
Full Text Sources
Other Literature Sources
Molecular Biology Databases
Miscellaneous