Synaptotagmin-12, a synaptic vesicle phosphoprotein that modulates spontaneous neurotransmitter release
- PMID: 17190793
- PMCID: PMC2063632
- DOI: 10.1083/jcb.200607021
Synaptotagmin-12, a synaptic vesicle phosphoprotein that modulates spontaneous neurotransmitter release
Abstract
Central synapses exhibit spontaneous neurotransmitter release that is selectively regulated by cAMP-dependent protein kinase A (PKA). We now show that synaptic vesicles contain synaptotagmin-12, a synaptotagmin isoform that differs from classical synaptotagmins in that it does not bind Ca(2+). In synaptic vesicles, synaptotagmin-12 forms a complex with synaptotagmin-1 that prevents synaptotagmin-1 from interacting with SNARE complexes. We demonstrate that synaptotagmin-12 is phosphorylated by cAMP-dependent PKA on serine(97), and show that expression of synaptotagmin-12 in neurons increases spontaneous neurotransmitter release by approximately threefold, but has no effect on evoked release. Replacing serine(97) by alanine abolishes synaptotagmin-12 phosphorylation and blocks its effect on spontaneous release. Our data suggest that spontaneous synaptic-vesicle exocytosis is selectively modulated by a Ca(2+)-independent synaptotagmin isoform, synaptotagmin-12, which is controlled by cAMP-dependent phosphorylation.
Figures
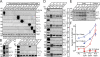
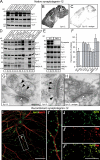
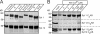
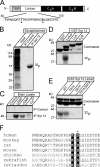
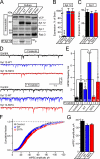
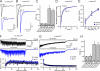
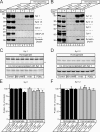
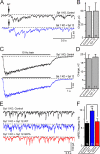
Similar articles
-
Close membrane-membrane proximity induced by Ca(2+)-dependent multivalent binding of synaptotagmin-1 to phospholipids.Nat Struct Mol Biol. 2006 Mar;13(3):209-17. doi: 10.1038/nsmb1056. Epub 2006 Feb 19. Nat Struct Mol Biol. 2006. PMID: 16491093
-
Synaptotagmin-1 and -7 Are Redundantly Essential for Maintaining the Capacity of the Readily-Releasable Pool of Synaptic Vesicles.PLoS Biol. 2015 Oct 5;13(10):e1002267. doi: 10.1371/journal.pbio.1002267. eCollection 2015 Oct. PLoS Biol. 2015. PMID: 26437117 Free PMC article.
-
Allosteric stabilization of calcium and phosphoinositide dual binding engages several synaptotagmins in fast exocytosis.Elife. 2022 Aug 5;11:e74810. doi: 10.7554/eLife.74810. Elife. 2022. PMID: 35929728 Free PMC article.
-
Unraveling the mechanisms of synaptotagmin and SNARE function in neurotransmitter release.Trends Cell Biol. 2006 Jul;16(7):339-50. doi: 10.1016/j.tcb.2006.04.006. Epub 2006 May 12. Trends Cell Biol. 2006. PMID: 16698267 Review.
-
How does synaptotagmin trigger neurotransmitter release?Annu Rev Biochem. 2008;77:615-41. doi: 10.1146/annurev.biochem.77.062005.101135. Annu Rev Biochem. 2008. PMID: 18275379 Review.
Cited by
-
A resting pool of vesicles is responsible for spontaneous vesicle fusion at the synapse.Nat Neurosci. 2009 Jun;12(6):751-8. doi: 10.1038/nn.2317. Epub 2009 May 10. Nat Neurosci. 2009. PMID: 19430474 Free PMC article.
-
Quantitative comparison of glutamatergic and GABAergic synaptic vesicles unveils selectivity for few proteins including MAL2, a novel synaptic vesicle protein.J Neurosci. 2010 Jan 6;30(1):2-12. doi: 10.1523/JNEUROSCI.4074-09.2010. J Neurosci. 2010. PMID: 20053882 Free PMC article.
-
Potential roles of synaptotagmin family members in cancers: Recent advances and prospects.Front Med (Lausanne). 2022 Aug 8;9:968081. doi: 10.3389/fmed.2022.968081. eCollection 2022. Front Med (Lausanne). 2022. PMID: 36004367 Free PMC article. Review.
-
Comprehensive integrative analyses identify GLT8D1 and CSNK2B as schizophrenia risk genes.Nat Commun. 2018 Feb 26;9(1):838. doi: 10.1038/s41467-018-03247-3. Nat Commun. 2018. PMID: 29483533 Free PMC article.
-
Dendritic SNAREs add a new twist to the old neuron theory.Proc Natl Acad Sci U S A. 2011 Nov 29;108(48):19113-20. doi: 10.1073/pnas.1017235108. Epub 2011 Nov 11. Proc Natl Acad Sci U S A. 2011. PMID: 22080607 Free PMC article. Review.
References
-
- Angleson, J.K., and W.J. Betz. 2001. Intraterminal Ca(2+) and spontaneous transmitter release at the frog neuromuscular junction. J. Neurophysiol. 85:287–294. - PubMed
-
- Banerjee, A., J.A. Kowalchyk, B.R. DasGupta, and T.F. Martin. 1996. SNAP-25 is required for a late postdocking step in Ca2+-dependent exocytosis. J. Biol. Chem. 271:20227–20230. - PubMed
-
- Bennett, M.K., N. Calakos, and R.H. Scheller. 1992. Syntaxin: a synaptic protein implicated in docking of synaptic vesicles at presynaptic active zones. Science. 257:255–259. - PubMed
MeSH terms
Substances
LinkOut - more resources
Full Text Sources
Molecular Biology Databases
Miscellaneous