Promyelocytic leukemia nuclear bodies behave as DNA damage sensors whose response to DNA double-strand breaks is regulated by NBS1 and the kinases ATM, Chk2, and ATR
- PMID: 17030982
- PMCID: PMC2064496
- DOI: 10.1083/jcb.200604009
Promyelocytic leukemia nuclear bodies behave as DNA damage sensors whose response to DNA double-strand breaks is regulated by NBS1 and the kinases ATM, Chk2, and ATR
Abstract
The promyelocytic leukemia (PML) nuclear body (NB) is a dynamic subnuclear compartment that is implicated in tumor suppression, as well as in the transcription, replication, and repair of DNA. PML NB number can change during the cell cycle, increasing in S phase and in response to cellular stress, including DNA damage. Although topological changes in chromatin after DNA damage may affect the integrity of PML NBs, the molecular or structural basis for an increase in PML NB number has not been elucidated. We demonstrate that after DNA double-strand break induction, the increase in PML NB number is based on a biophysical process, as well as ongoing cell cycle progression and DNA repair. PML NBs increase in number by a supramolecular fission mechanism similar to that observed in S-phase cells, and which is delayed or inhibited by the loss of function of NBS1, ATM, Chk2, and ATR kinase. Therefore, an increase in PML NB number is an intrinsic element of the cellular response to DNA damage.
Figures
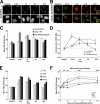
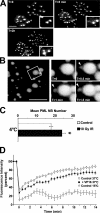
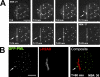
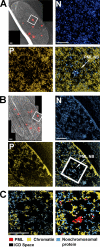
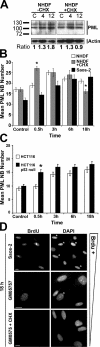
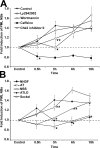
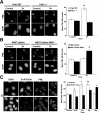
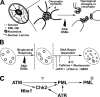
Similar articles
-
KAP1 depletion increases PML nuclear body number in concert with ultrastructural changes in chromatin.Cell Cycle. 2011 Jan 15;10(2):308-22. doi: 10.4161/cc.10.2.14551. Epub 2011 Jan 15. Cell Cycle. 2011. PMID: 21228624
-
E2F1 uses the ATM signaling pathway to induce p53 and Chk2 phosphorylation and apoptosis.Mol Cancer Res. 2004 Apr;2(4):203-14. Mol Cancer Res. 2004. PMID: 15140942
-
Roles of ATM and NBS1 in chromatin structure modulation and DNA double-strand break repair.Nat Cell Biol. 2007 Jun;9(6):683-90. doi: 10.1038/ncb1599. Epub 2007 May 7. Nat Cell Biol. 2007. PMID: 17486112
-
[A cell and genotoxic stress: a reaction to double strand breaks of DNA].Cas Lek Cesk. 2005;144 Suppl 3:13-7. Cas Lek Cesk. 2005. PMID: 16335257 Review. Czech.
-
Structure, dynamics and functions of promyelocytic leukaemia nuclear bodies.Nat Rev Mol Cell Biol. 2007 Dec;8(12):1006-16. doi: 10.1038/nrm2277. Nat Rev Mol Cell Biol. 2007. PMID: 17928811 Review.
Cited by
-
PML is required for telomere stability in non-neoplastic human cells.Oncogene. 2016 Apr 7;35(14):1811-21. doi: 10.1038/onc.2015.246. Epub 2015 Jun 29. Oncogene. 2016. PMID: 26119943 Free PMC article.
-
The MORC family: new epigenetic regulators of transcription and DNA damage response.Epigenetics. 2013 Jul;8(7):685-93. doi: 10.4161/epi.24976. Epub 2013 May 17. Epigenetics. 2013. PMID: 23804034 Free PMC article.
-
Dynamic PML protein nucleolar associations with persistent DNA damage lesions in response to nucleolar stress and senescence-inducing stimuli.Aging (Albany NY). 2019 Sep 7;11(17):7206-7235. doi: 10.18632/aging.102248. Epub 2019 Sep 7. Aging (Albany NY). 2019. PMID: 31493766 Free PMC article.
-
Promyelocytic leukemia bodies tether to early endosomes during mitosis.Cell Cycle. 2014;13(11):1749-55. doi: 10.4161/cc.28653. Epub 2014 Mar 26. Cell Cycle. 2014. PMID: 24675887 Free PMC article.
-
The intrinsic antiviral defense to incoming HSV-1 genomes includes specific DNA repair proteins and is counteracted by the viral protein ICP0.PLoS Pathog. 2011 Jun;7(6):e1002084. doi: 10.1371/journal.ppat.1002084. Epub 2011 Jun 16. PLoS Pathog. 2011. PMID: 21698222 Free PMC article.
References
-
- Arienti, K.L., A. Brunmark, F.U. Axe, K. McClure, A. Lee, J. Blevitt, D.K. Neff, L. Huang, S. Crawford, C.R. Pandit, et al. 2005. Checkpoint kinase inhibitors: SAR and radioprotective properties of a series of 2-arylbenzimidazoles. J. Med. Chem. 48:1873–1885. - PubMed
-
- Bernardi, R., P.P. Scaglioni, S. Bergmann, H.F. Horn, K.H. Vousden, and P.P. Pandolfi. 2004. PML regulates p53 stability by sequestering Mdm2 to the nucleolus. Nat. Cell Biol. 6:665–672. - PubMed
-
- Bradshaw, P.S., D.J. Stavropoulos, and M.S. Meyn. 2005. Human telomeric protein TRF2 associates with genomic double-strand breaks as an early response to DNA damage. Nat. Genet. 37:193–197. - PubMed
-
- Bristow, R.G., and R.P. Hill. 2005. Molecular and cellular basis of radiotherapy. In The Basic Science of Oncology, 4th edition. I.F. Tannock, R.P. Hill, R.G. Bristow, and L. Harrington, editors. McGraw-Hill, New York. 261–288.
Publication types
MeSH terms
Substances
LinkOut - more resources
Full Text Sources
Other Literature Sources
Molecular Biology Databases
Research Materials
Miscellaneous