Characterization of a proton-activated, outwardly rectifying anion channel
- PMID: 15961423
- PMCID: PMC1474181
- DOI: 10.1113/jphysiol.2005.089888
Characterization of a proton-activated, outwardly rectifying anion channel
Abstract
Anion channels are present in every mammalian cell and serve many different functions, including cell volume regulation, ion transport across epithelia, regulation of membrane potential and vesicular acidification. Here we characterize a proton-activated, outwardly rectifying current endogenously expressed in HEK293 cells. Binding of three to four protons activated the anion permeable channels at external pH below 5.5 (50% activation at pH 5.1). The proton-activated current is strongly outwardly rectifying, due to an outwardly rectifying single channel conductance and an additional voltage dependent facilitation at depolarized membrane potentials. The anion channel blocker 4,4'-diisothiocyanostilbene-2,2'-disulphonic acid (DIDS) rapidly and potently inhibited the channel (IC50: 2.9 microm). Flufenamic acid blocked this channel only slowly, while mibefradil and amiloride at high concentrations had no effect. As determined from reversal potential measurements under bi-ionic conditions, the relative permeability sequence of this channel was SCN-> I-> NO3-> Br-> Cl-. None of the previously characterized anion channel matches the properties of the proton-activated, outwardly rectifying channel. Specifically, the proton-activated and the volume-regulated anion channels are two distinct and separable populations of ion channels, each having its own set of biophysical and pharmacological properties. We also demonstrate endogenous proton-activated currents in primary cultured hippocampal astrocytes. The proton-activated current in astrocytes is also carried by anions, strongly outwardly rectifying, voltage dependent and inhibited by DIDS. Proton-activated, outwardly rectifying anion channels therefore may be a broadly expressed part of the anionic channel repertoire of mammalian cells.
Figures
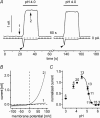
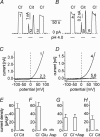
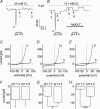
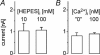
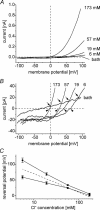
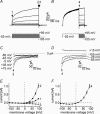
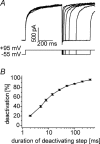
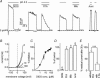
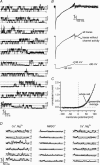
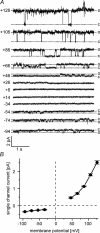
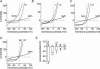
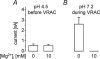
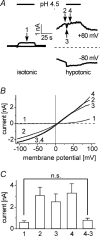
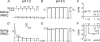
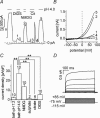
Similar articles
-
Volume-activated chloride currents in interstitial cells of Cajal.Am J Physiol Gastrointest Liver Physiol. 2005 Nov;289(5):G791-7. doi: 10.1152/ajpgi.00050.2005. Am J Physiol Gastrointest Liver Physiol. 2005. PMID: 16227526
-
Characterization of Ca2+-activated Cl- currents in mouse kidney inner medullary collecting duct cells.Am J Physiol Renal Physiol. 2003 Aug;285(2):F326-35. doi: 10.1152/ajprenal.00034.2003. Epub 2003 Apr 29. Am J Physiol Renal Physiol. 2003. PMID: 12724129
-
A role for the volume regulated anion channel in volume regulation in the murine CNS cell line, CAD.Acta Physiol (Oxf). 2010 Feb;198(2):159-68. doi: 10.1111/j.1748-1716.2009.02050.x. Epub 2009 Oct 7. Acta Physiol (Oxf). 2010. PMID: 19811460
-
Hypotonicity and ethanol modulate BK channel activity and chloride currents in GH4/C1 pituitary tumour cells.Acta Physiol (Oxf). 2006 May-Jun;187(1-2):51-9. doi: 10.1111/j.1748-1716.2006.01544.x. Acta Physiol (Oxf). 2006. PMID: 16734742 Review.
-
Mechanism of anion permeation through channels gated by glycine and gamma-aminobutyric acid in mouse cultured spinal neurones.J Physiol. 1987 Apr;385:243-86. doi: 10.1113/jphysiol.1987.sp016493. J Physiol. 1987. PMID: 2443667 Free PMC article. Review.
Cited by
-
Kinetic analysis of the effects of H+ or Ni2+ on Kv1.5 current shows that both ions enhance slow inactivation and induce resting inactivation.J Physiol. 2010 Aug 15;588(Pt 16):3011-30. doi: 10.1113/jphysiol.2010.191544. Epub 2010 Jun 25. J Physiol. 2010. PMID: 20581043 Free PMC article.
-
Molecular determinants of pH sensing in the proton-activated chloride channel.Proc Natl Acad Sci U S A. 2022 Aug 2;119(31):e2200727119. doi: 10.1073/pnas.2200727119. Epub 2022 Jul 25. Proc Natl Acad Sci U S A. 2022. PMID: 35878032 Free PMC article.
-
The molecular basis for species-specific activation of human TRPA1 protein by protons involves poorly conserved residues within transmembrane domains 5 and 6.J Biol Chem. 2013 Jul 12;288(28):20280-92. doi: 10.1074/jbc.M113.479337. Epub 2013 May 24. J Biol Chem. 2013. PMID: 23709225 Free PMC article.
-
To Be or Not to Be an Ion Channel: Cryo-EM Structures Have a Say.Cells. 2023 Jul 17;12(14):1870. doi: 10.3390/cells12141870. Cells. 2023. PMID: 37508534 Free PMC article. Review.
-
Ion permeation controlled by hydrophobic residues and proton binding in the proton-activated chloride channel.iScience. 2021 Nov 1;24(12):103395. doi: 10.1016/j.isci.2021.103395. eCollection 2021 Dec 17. iScience. 2021. PMID: 34825147 Free PMC article.
References
-
- Accardi A, Miller C. Secondary active transport mediated by a prokaryotic homologue of ClC Cl− channels. Nature. 2004;427:803–807. - PubMed
-
- Ando-Akatsuka Y, Abdullaev IF, Lee EL, Okada Y, Sabirov RZ. Down-regulation of volume-sensitive Cl− channels by CFTR is mediated by the second nucleotide-binding domain. Pflügers Arch. 2002;445:177–186. - PubMed
-
- Auzanneau C, Thoreau V, Kitzis A, Becq F. A novel voltage dependent chloride current activated by extracellular acidic pH in cultured rat Sertoli cells. J Biol Chem. 2003;278:19230–19236. - PubMed
-
- Blaustein MP, Lederer WJ. Sodium/calcium exchange: its physiological implications. Physiol Rev. 1999;79:763–854. - PubMed
Publication types
MeSH terms
Substances
LinkOut - more resources
Full Text Sources
Research Materials