Myosin-1a is critical for normal brush border structure and composition
- PMID: 15758024
- PMCID: PMC1087248
- DOI: 10.1091/mbc.e04-12-1116
Myosin-1a is critical for normal brush border structure and composition
Abstract
To develop our understanding of myosin-1a function in vivo, we have created a mouse line null for the myosin-1a gene. Myosin-1a knockout mice demonstrate no overt phenotypes at the whole animal level but exhibit significant perturbations and signs of stress at the cellular level. Among these are defects in microvillar membrane morphology, distinct changes in brush-border organization, loss of numerous cytoskeletal and membrane components from the brush border, and redistribution of intermediate filament proteins into the brush border. We also observed significant ectopic recruitment of another short-tailed class I motor, myosin-1c, into the brush border of knockout enterocytes. This latter finding, a clear demonstration of functional redundancy among vertebrate myosins-I, may account for the lack of a whole animal phenotype. Nevertheless, these results indicate that myosin-1a is a critical multifunctional component of the enterocyte, required for maintaining the normal composition and highly ordered structure of the brush border.
Figures
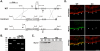
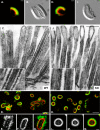
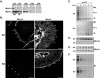
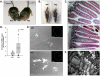
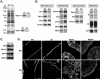
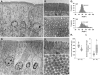
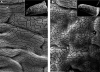
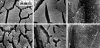
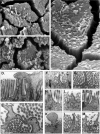
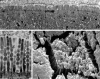
Comment in
-
An MBoC favorite: Myosin-1a is critical for normal brush border structure and composition.Mol Biol Cell. 2012 Jul;23(13):2401. doi: 10.1091/mbc.E12-03-0188. Mol Biol Cell. 2012. PMID: 22745338 Free PMC article. No abstract available.
Similar articles
-
An MBoC favorite: Myosin-1a is critical for normal brush border structure and composition.Mol Biol Cell. 2012 Jul;23(13):2401. doi: 10.1091/mbc.E12-03-0188. Mol Biol Cell. 2012. PMID: 22745338 Free PMC article. No abstract available.
-
Differential localization and dynamics of class I myosins in the enterocyte microvillus.Mol Biol Cell. 2010 Mar 15;21(6):970-8. doi: 10.1091/mbc.e09-07-0638. Epub 2010 Jan 20. Mol Biol Cell. 2010. PMID: 20089841 Free PMC article.
-
Myosin 5b is required for proper localization of the intermicrovillar adhesion complex in the intestinal brush border.Am J Physiol Gastrointest Liver Physiol. 2022 Nov 1;323(5):G501-G510. doi: 10.1152/ajpgi.00212.2022. Epub 2022 Oct 11. Am J Physiol Gastrointest Liver Physiol. 2022. PMID: 36218265 Free PMC article.
-
Shaping the intestinal brush border.J Cell Biol. 2014 Nov 24;207(4):441-51. doi: 10.1083/jcb.201407015. J Cell Biol. 2014. PMID: 25422372 Free PMC article. Review.
-
Lipid raft organization and function in brush borders of epithelial cells.Mol Membr Biol. 2006 Jan-Feb;23(1):71-9. doi: 10.1080/09687860500445604. Mol Membr Biol. 2006. PMID: 16611582 Review.
Cited by
-
Ready…aim…fire into the lumen: a new role for enterocyte microvilli in gut host defense.Gut Microbes. 2012 Sep-Oct;3(5):460-2. doi: 10.4161/gmic.21247. Epub 2012 Jul 24. Gut Microbes. 2012. PMID: 22825496 Free PMC article. Review.
-
Myosin 1G is an abundant class I myosin in lymphocytes whose localization at the plasma membrane depends on its ancient divergent pleckstrin homology (PH) domain (Myo1PH).J Biol Chem. 2010 Mar 19;285(12):8675-86. doi: 10.1074/jbc.M109.086959. Epub 2010 Jan 12. J Biol Chem. 2010. PMID: 20071333 Free PMC article.
-
Leveraging the membrane - cytoskeleton interface with myosin-1.Trends Cell Biol. 2010 Jul;20(7):418-26. doi: 10.1016/j.tcb.2010.04.004. Epub 2010 May 12. Trends Cell Biol. 2010. PMID: 20471271 Free PMC article. Review.
-
MYO1H is a novel candidate gene for autosomal dominant pure hereditary spastic paraplegia.Mol Genet Genomics. 2022 Jul;297(4):1141-1150. doi: 10.1007/s00438-022-01910-5. Epub 2022 Jun 15. Mol Genet Genomics. 2022. PMID: 35704118
-
Lessons Learned from Large-Scale, First-Tier Clinical Exome Sequencing in a Highly Consanguineous Population.Am J Hum Genet. 2019 Jun 6;104(6):1182-1201. doi: 10.1016/j.ajhg.2019.04.011. Epub 2019 May 23. Am J Hum Genet. 2019. PMID: 31130284 Free PMC article.
References
-
- Altman, D., Sweeney, H. L., and Spudich, J. A. (2004). The mechanism of myosin VI translocation and its load-induced anchoring. Cell 116, 737-749. - PubMed
-
- Braccia, A., Villani, M., Immerdal, L., Niels-Christiansen, L. L., Nystrom, B. T., Hansen, G. H., and Danielsen, E. M. (2003). Microvillar membrane microdomains exist at physiological temperature. Role of galectin-4 as lipid raft stabilizer revealed by “superrafts.” J. Biol. Chem. 278, 15679-15684. - PubMed
-
- Brown, D. A., and London, E. (1998). Functions of lipid rafts in biological membranes. Annu. Rev. Cell Dev. Biol. 14, 111-136. - PubMed
Publication types
MeSH terms
Substances
Grants and funding
LinkOut - more resources
Full Text Sources
Other Literature Sources
Molecular Biology Databases