Metabolic interdependence of obligate intracellular bacteria and their insect hosts
- PMID: 15590782
- PMCID: PMC539007
- DOI: 10.1128/MMBR.68.4.745-770.2004
Metabolic interdependence of obligate intracellular bacteria and their insect hosts
Abstract
Mutualistic associations of obligate intracellular bacteria and insects have attracted much interest in the past few years due to the evolutionary consequences for their genome structure. However, much less attention has been paid to the metabolic ramifications for these endosymbiotic microorganisms, which have to compete with but also to adapt to another metabolism--that of the host cell. This review attempts to provide insights into the complex physiological interactions and the evolution of metabolic pathways of several mutualistic bacteria of aphids, ants, and tsetse flies and their insect hosts.
Figures
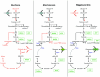
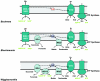
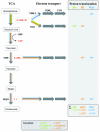
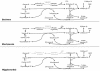
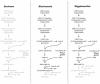
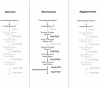
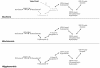
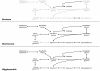
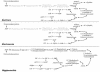
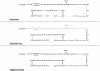
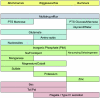
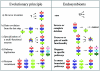
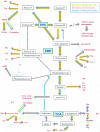
Similar articles
-
Genome evolution in bacterial endosymbionts of insects.Nat Rev Genet. 2002 Nov;3(11):850-61. doi: 10.1038/nrg931. Nat Rev Genet. 2002. PMID: 12415315 Review.
-
Reductive evolution of bacterial genome in insect gut environment.Genome Biol Evol. 2011;3:702-14. doi: 10.1093/gbe/evr064. Epub 2011 Jul 6. Genome Biol Evol. 2011. PMID: 21737395 Free PMC article.
-
Evolutionary dynamics of insect symbiont associations.Trends Ecol Evol. 2007 Dec;22(12):625-7. doi: 10.1016/j.tree.2007.08.013. Epub 2007 Nov 5. Trends Ecol Evol. 2007. PMID: 17981362
-
Insect symbionts as hidden players in insect-plant interactions.Trends Ecol Evol. 2012 Dec;27(12):705-11. doi: 10.1016/j.tree.2012.08.013. Epub 2012 Sep 15. Trends Ecol Evol. 2012. PMID: 22985943 Review.
-
Genomics of intracellular symbionts in insects.Int J Med Microbiol. 2010 Jun;300(5):271-8. doi: 10.1016/j.ijmm.2009.12.001. Epub 2010 Jan 25. Int J Med Microbiol. 2010. PMID: 20093081 Review.
Cited by
-
A global characterization and identification of multifunctional enzymes.PLoS One. 2012;7(6):e38979. doi: 10.1371/journal.pone.0038979. Epub 2012 Jun 18. PLoS One. 2012. PMID: 22723914 Free PMC article.
-
Microbial lifestyle and genome signatures.Curr Genomics. 2012 Apr;13(2):153-62. doi: 10.2174/138920212799860698. Curr Genomics. 2012. PMID: 23024607 Free PMC article.
-
Metabolic classification of microbial genomes using functional probes.BMC Genomics. 2012 Apr 27;13:157. doi: 10.1186/1471-2164-13-157. BMC Genomics. 2012. PMID: 22537274 Free PMC article.
-
Computational prediction of genomic functional cores specific to different microbes.J Mol Evol. 2006 Dec;63(6):733-46. doi: 10.1007/s00239-005-0250-9. Epub 2006 Nov 10. J Mol Evol. 2006. PMID: 17103060
-
Unique trajectory of gene family evolution from genomic analysis of nearly all known species in an ancient yeast lineage.bioRxiv [Preprint]. 2024 Jun 6:2024.06.05.597512. doi: 10.1101/2024.06.05.597512. bioRxiv. 2024. PMID: 38895429 Free PMC article. Preprint.
References
-
- Adams, M. D., S. E. Celniker, R. A. Holt, C. A. Evans, J. D. Gocayne, P. G. Amanatides, S. E. Scherer, P. W. Li, R. A. Hoskins, R. F. Galle, R. A. George, S. E. Lewis, S. Richards, M. Ashburner, S. N. Henderson, G. G. Sutton, J. R. Wortman, M. D. Yandell, Q. Zhang, L. X. Chen, R. C. Brandon, Y. H. Rogers, R. G. Blazej, M. Champe, B. D. Pfeiffer, K. H. Wan, C. Doyle, E. G. Baxter, G. Helt, C. R. Nelson, G. L. Gabor, J. F. Abril, A. Agbayani, H. J. An, C. Andrews-Pfannkoch, D. Baldwin, R. M. Ballew, A. Basu, J. Baxendale, L. Bayraktaroglu, E. M. Beasley, K. Y. Beeson, P. V. Benos, B. P. Berman, D. Bhandari, S. Bolshakov, D. Borkova, M. R. Botchan, J. Bouck, P. Brokstein, P. Brottier, K. C. Burtis, D. A. Busam, H. Butler, E. Cadieu, A. Center, I. Chandra, J. M. Cherry, S. Cawley, C. Dahlke, L. B. Davenport, P. Davies, B. de Pablos, A. Delcher, Z. Deng, A. D. Mays, I. Dew, S. M. Dietz, K. Dodson, L. E. Doup, M. Downes, S. Dugan-Rocha, B. C. Dunkov, P. Dunn, K. J. Durbin, C. C. Evangelista, C. Ferraz, S. Ferriera, W. Fleischmann, C. Fosler, A. E. Gabrielian, N. S. Garg, W. M. Gelbart, K. Glasser, A. Glodek, F. Gong, J. H. Gorrell, Z. Gu, P. Guan, M. Harris, N. L. Harris, D. Harvey, T. J. Heiman, J. R. Hernandez, J. Houck, D. Hostin, K. A. Houston, T. J. Howland, M. H. Wei, C. Ibegwam, M. Jalali, F. Kalush, G. H. Karpen, Z. Ke, J. A. Kennison, K. A. Ketchum, B. E. Kimmel, C. D. Kodira, C. Kraft, S. Kravitz, D. Kulp, Z. Lai, P. Lasko, Y. Lei, A. A. Levitsky, J. Li, Z. Li, Y. Liang, X. Lin, X. Liu, B. Mattei, T. C. McIntosh, M. P. McLeod, D. McPherson, G. Merkulov, N. V. Milshina, C. Mobarry, J. Morris, A. Moshrefi, S. M. Mount, M. Moy, B. Murphy, L. Murphy, D. M. Muzny, D. L. Nelson, D. R. Nelson, K. A. Nelson, K. Nixon, D. R. Nusskern, J. M. Pacleb, M. Palazzolo, G. S. Pittman, S. Pan, J. Pollard, V. Puri, M. G. Reese, K. Reinert, K. Remington, R. D. Saunders, F. Scheeler, H. Shen, B. C. Shue, I. Siden-Kiamos, M. Simpson, M. P. Skupski, T. Smith, E. Spier, A. C. Spradling, M. Stapleton, R. Strong, E. Sun, R. Svirskas, C. Tector, R. Turner, E. Venter, A. H. Wang, X. Wang, Z. Y. Wang, D. A. Wassarman, G. M. Weinstock, J. Weissenbach, S. M. Williams, T. Woodage, K. C. Worley, D. Wu, S. Yang, Q. A. Yao, J. Ye, R. F. Yeh, J. S. Zaveri, M. Zhan, G. Zhang, Q. Zhao, L. Zheng, X. H. Zheng, F. N. Zhong, W. Zhong, X. Zhou, S. Zhu, X. Zhu, H. O. Smith, R. A. Gibbs, E. W. Myers, G. M. Rubin and J. C. Venter. 2000. The genome sequence of Drosophila melanogaster. Science 287:2185-2195. - PubMed
-
- Akman, L., A. Yamashita, H. Watanabe, K. Oshima, T. Shiba, M. Hattori, and S. Aksoy. 2002. Genome sequence of the endocellular obligate symbiont of tsetse flies, Wigglesworthia glossinidia. Nat. Genet. 32:402-407. - PubMed
-
- Allikmets, R., B. Gerrard, D. Court, and M. Dean. 1993. Cloning and organization of the abc and mdl genes of Escherichia coli: relationship to eukaryotic multidrug resistance. Gene 136:231-236. - PubMed
-
- Andersson, S. G. E., A. Zomorodipour, J. O. Andersson, T. Sicheritz-Ponten, U. C. M. Alsmark, R. M. Podowski, A. K. Naslund, A. S. Eriksson, H. H. Winkler, and C. G. Kurland. 1998. The genome sequence of Rickettsia prowazekii and the origin of mitochondria. Nature 396:133-140. - PubMed
-
- Barancin, C. E., J. C. Smoot, R. H. Findlkay, and L. A. Actis. 1998. Plasmid- mediated histamine biosynthesis in the bacterial fish pathogen Vibrio anguillarum. Plasmid 39:235-244. - PubMed
Publication types
MeSH terms
LinkOut - more resources
Full Text Sources
Miscellaneous