The GTPase ARF1p controls the sequence-specific vacuolar sorting route to the lytic vacuole
- PMID: 12724547
- PMCID: PMC153729
- DOI: 10.1105/tpc.010140
The GTPase ARF1p controls the sequence-specific vacuolar sorting route to the lytic vacuole
Abstract
We have studied the transport of soluble cargo molecules by inhibiting specific transport steps to and from the Golgi apparatus. Inhibition of export from the Golgi via coexpression of a dominant-negative GTP-restricted ARF1 mutant (Q71L) inhibits the secretion of alpha-amylase and simultaneously induces the secretion of the vacuolar protein phytepsin to the culture medium. By contrast, specific inhibition of endoplasmic reticulum export via overexpression of Sec12p or coexpression of a GTP-restricted form of Sar1p inhibits the anterograde transport of either cargo molecule in a similar manner. Increased secretion of the vacuolar protein was not observed after incubation with the drug brefeldin A or after coexpression of the GDP-restricted mutant of ARF1 (T31N). Therefore, the differential effect of inducing the secretion of one cargo molecule while inhibiting the secretion of another is dependent on the GTP hydrolysis by ARF1p and is not caused by a general inhibition of Golgi-derived COPI vesicle traffic. Moreover, we demonstrate that GTP-restricted ARF1-stimulated secretion is observed only for cargo molecules that are expected to be sorted in a BP80-dependent manner, exhibiting sequence-specific, context-independent, vacuolar sorting signals. Induced secretion of proteins carrying C-terminal vacuolar sorting signals was not observed. This finding suggests that ARF1p influences the BP80-mediated transport route to the vacuole in addition to transport steps of the default secretory pathway to the cell surface.
Figures
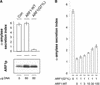
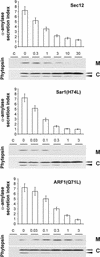
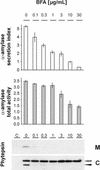
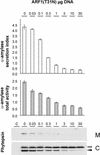
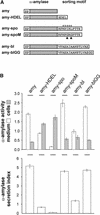
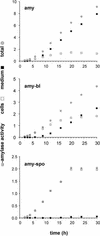
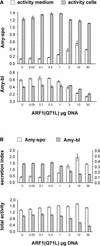
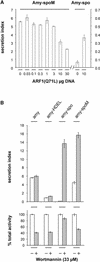
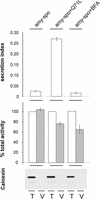
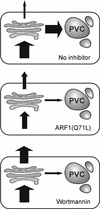
Similar articles
-
COPI vesicles accumulating in the presence of a GTP restricted arf1 mutant are depleted of anterograde and retrograde cargo.J Cell Sci. 2000 Jan;113 ( Pt 1):135-44. doi: 10.1242/jcs.113.1.135. J Cell Sci. 2000. PMID: 10591632
-
Dissection of COPI and Arf1 dynamics in vivo and role in Golgi membrane transport.Nature. 2002 May 9;417(6885):187-93. doi: 10.1038/417187a. Nature. 2002. PMID: 12000962
-
A vacuolar sorting domain may also influence the way in which proteins leave the endoplasmic reticulum.Plant Cell. 2001 Sep;13(9):2021-32. doi: 10.1105/tpc.000533. Plant Cell. 2001. PMID: 11549761 Free PMC article.
-
The COPI system: molecular mechanisms and function.FEBS Lett. 2009 Sep 3;583(17):2701-9. doi: 10.1016/j.febslet.2009.07.032. Epub 2009 Jul 22. FEBS Lett. 2009. PMID: 19631211 Review.
-
Traffic COPs of the early secretory pathway.Traffic. 2000 May;1(5):371-7. doi: 10.1034/j.1600-0854.2000.010501.x. Traffic. 2000. PMID: 11208122 Review.
Cited by
-
Mechanisms and concepts paving the way towards a complete transport cycle of plant vacuolar sorting receptors.Plant Cell. 2012 May;24(5):1714-32. doi: 10.1105/tpc.112.095679. Epub 2012 May 8. Plant Cell. 2012. PMID: 22570446 Free PMC article. Review.
-
The Coordinated KNR6-AGAP-ARF1 Complex Modulates Vegetative and Reproductive Traits by Participating in Vesicle Trafficking in Maize.Cells. 2021 Sep 30;10(10):2601. doi: 10.3390/cells10102601. Cells. 2021. PMID: 34685581 Free PMC article.
-
Oryzalin bodies: in addition to its anti-microtubule properties, the dinitroaniline herbicide oryzalin causes nodulation of the endoplasmic reticulum.Protoplasma. 2009 Jul;236(1-4):73-84. doi: 10.1007/s00709-009-0059-2. Epub 2009 Jun 26. Protoplasma. 2009. PMID: 19557498
-
Is the 6 kDa tobacco etch viral protein a bona fide ERES marker?J Exp Bot. 2011 Oct;62(14):5013-23. doi: 10.1093/jxb/err200. Epub 2011 Jun 24. J Exp Bot. 2011. PMID: 21705387 Free PMC article.
-
Studying Secretory Protein Synthesis in Nicotiana benthamiana Protoplasts.Methods Mol Biol. 2024;2772:391-405. doi: 10.1007/978-1-0716-3710-4_30. Methods Mol Biol. 2024. PMID: 38411831
References
-
- Ahmed, S.U., Rojo, E., Kovaleva, V., Venkataraman, S., Dombrowski, J.E., Matsuoka, K., and Raikhel, N.V. (2000). The plant vacuolar sorting receptor AtELP is involved in transport of NH(2)-terminal propeptide-containing vacuolar proteins in Arabidopsis thaliana. J. Cell Biol. 149, 1335–1344. - PMC - PubMed
-
- Barlowe, C., Orci, L., Yeung, T., Hosobuchi, M., Hamamoto, S., Salama, N., Rexach, M.F., Ravazzola, M., Amherdt, M., and Schekman, R. (1994). COPII: A membrane coat formed by Sec proteins that drive vesicle budding from the endoplasmic reticulum. Cell 77, 895–907. - PubMed
-
- Bassham, D.C., and Raikhel, N.V. (2000). Unique features of the plant vacuolar sorting machinery. Curr. Opin. Cell Biol. 12, 491–495. - PubMed
Publication types
MeSH terms
Substances
LinkOut - more resources
Full Text Sources
Other Literature Sources