Mitochondrial targeting and a novel transmembrane arrest of Alzheimer's amyloid precursor protein impairs mitochondrial function in neuronal cells
- PMID: 12695498
- PMCID: PMC2172865
- DOI: 10.1083/jcb.200207030
Mitochondrial targeting and a novel transmembrane arrest of Alzheimer's amyloid precursor protein impairs mitochondrial function in neuronal cells
Abstract
Alzheimer's amyloid precursor protein 695 (APP) is a plasma membrane protein, which is known to be the source of the toxic amyloid beta (Abeta) peptide associated with the pathogenesis of Alzheimer's disease (AD). Here we demonstrate that by virtue of its chimeric NH2-terminal signal, APP is also targeted to mitochondria of cortical neuronal cells and select regions of the brain of a transgenic mouse model for AD. The positively charged residues at 40, 44, and 51 of APP are critical components of the mitochondrial-targeting signal. Chemical cross-linking together with immunoelectron microscopy show that the mitochondrial APP exists in NH2-terminal inside transmembrane orientation and in contact with mitochondrial translocase proteins. Mutational studies show that the acidic domain, which spans sequence 220-290 of APP, causes the transmembrane arrest with the COOH-terminal 73-kD portion of the protein facing the cytoplasmic side. Accumulation of full-length APP in the mitochondrial compartment in a transmembrane-arrested form, but not lacking the acidic domain, caused mitochondrial dysfunction and impaired energy metabolism. These results show, for the first time, that APP is targeted to neuronal mitochondria under some physiological and pathological conditions.
Figures
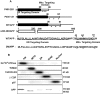
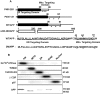
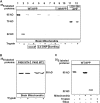
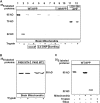
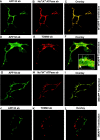
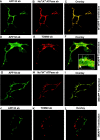
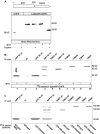
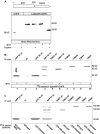
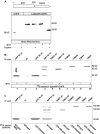
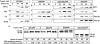
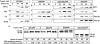
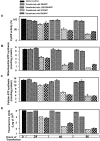
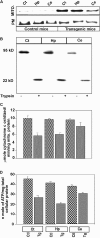
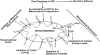
Similar articles
-
Mitochondria and vascular lesions as a central target for the development of Alzheimer's disease and Alzheimer disease-like pathology in transgenic mice.Neurol Res. 2003 Sep;25(6):665-74. doi: 10.1179/016164103101201977. Neurol Res. 2003. PMID: 14503022
-
Accumulation of amyloid precursor protein in the mitochondrial import channels of human Alzheimer's disease brain is associated with mitochondrial dysfunction.J Neurosci. 2006 Aug 30;26(35):9057-68. doi: 10.1523/JNEUROSCI.1469-06.2006. J Neurosci. 2006. PMID: 16943564 Free PMC article.
-
Mitochondrial dysfunction and accumulation of the β-secretase-cleaved C-terminal fragment of APP in Alzheimer's disease transgenic mice.Neurobiol Dis. 2012 Jan;45(1):417-24. doi: 10.1016/j.nbd.2011.09.001. Epub 2011 Sep 13. Neurobiol Dis. 2012. PMID: 21933711 Free PMC article.
-
Mitochondrial translocation of amyloid precursor protein and its cleaved products: relevance to mitochondrial dysfunction in Alzheimer's disease.Rev Neurosci. 2007;18(5):343-54. doi: 10.1515/revneuro.2007.18.5.343. Rev Neurosci. 2007. PMID: 19544621 Review.
-
Amyloid precursor protein and mitochondrial dysfunction in Alzheimer's disease.Neuroscientist. 2007 Dec;13(6):626-38. doi: 10.1177/1073858407303536. Epub 2007 Oct 2. Neuroscientist. 2007. PMID: 17911214 Review.
Cited by
-
Frontal cortical mitochondrial dysfunction and mitochondria-related β-amyloid accumulation by chronic sleep restriction in mice.Neuroreport. 2016 Aug 17;27(12):916-22. doi: 10.1097/WNR.0000000000000631. Neuroreport. 2016. PMID: 27341212 Free PMC article.
-
The Rationale for Insulin Therapy in Alzheimer's Disease.Molecules. 2016 May 26;21(6):689. doi: 10.3390/molecules21060689. Molecules. 2016. PMID: 27240327 Free PMC article. Review.
-
Biology of mitochondria in neurodegenerative diseases.Prog Mol Biol Transl Sci. 2012;107:355-415. doi: 10.1016/B978-0-12-385883-2.00005-9. Prog Mol Biol Transl Sci. 2012. PMID: 22482456 Free PMC article. Review.
-
DADS Analogues Ameliorated the Cognitive Impairments of Alzheimer-Like Rat Model Induced by Scopolamine.Neurotox Res. 2016 Oct;30(3):407-26. doi: 10.1007/s12640-016-9625-5. Epub 2016 May 5. Neurotox Res. 2016. PMID: 27149969
-
Mitochondrial targeting of mouse NQO1 and CYP1B1 proteins.Biochem Biophys Res Commun. 2013 Jun 14;435(4):727-32. doi: 10.1016/j.bbrc.2013.05.051. Epub 2013 May 18. Biochem Biophys Res Commun. 2013. PMID: 23692925 Free PMC article.
References
-
- Amuthan, G., G. Biswas, H.K. Anandatheerthavarada, C. Vijayasarathy, H.M. Shephard, and N.G. Avadhani. 2002. Mitochondrial stress-induced calcium signaling, phenotypic changes and invasive behavior in human lung carcinoma A549 cells. Oncogene. 21:7839–7849. - PubMed
-
- Anandatheerthavarada, H.K., G. Biswas, J. Mullick, N.B. Sepuri, L. Otvos, D. Pain, and N.G. Avadhani. 1999. Dual targeting of cytochrome P4502B1 to endoplasmic reticulum and mitochondria involves a novel signal activation by cyclic AMP-dependent phosphorylation at ser128. EMBO J. 18:5494–5504. - PMC - PubMed
-
- Beal, M.F. 1998. Mitochondrial dysfunction in neurodegenerative diseases. Biochim. Biophys. Acta. 1366:211–223. - PubMed
-
- Busciglio, J., A. Pelsman, C. Wong, G. Pigino, M. Yuan, H. Mori, and B.A. Yankner. 2002. Altered metabolism of the amyloid β precursor protein is associated with mitochondrial dysfunction in Down's syndrome. Neuron. 33:677–688. - PubMed
Publication types
MeSH terms
Substances
Grants and funding
LinkOut - more resources
Full Text Sources
Other Literature Sources
Medical
Miscellaneous