In Saccharomyces cerevisiae, ATP2 mRNA sorting to the vicinity of mitochondria is essential for respiratory function
- PMID: 12486010
- PMCID: PMC139110
- DOI: 10.1093/emboj/cdf690
In Saccharomyces cerevisiae, ATP2 mRNA sorting to the vicinity of mitochondria is essential for respiratory function
Abstract
We recently demonstrated that polysome-associated mRNAs that co-isolate with mitochondria encode a subset of mitochondrial proteins, and that the 3' UTRs of these transcripts are essential for their localization to the vicinity of the organelle. To address the question of the involvement of the mRNA targeting process in mitochondrial biogenesis, we studied the role of ATP2 3' UTR. An altered ATP2 allele in which the 3' UTR was replaced by the ADH1 3' UTR exhibits properties supporting the importance of mRNA localization to the vicinity of mitochondria: (i) the mutated strain presents a respiratory dysfunction; (ii) mitochondrial import of the protein translated from the altered gene is strongly reduced, even though the precursor is addressed to the organelle surface; (iii) systematic deletions of ATP2 3' UTR revealed a 100 nucleotide element presenting RNA targeting properties. Additionally, when the ATM1 3' UTR was replaced by the ADH1 3' UTR, we obtained cells in which ATM1 mRNA is also delocalized, and presenting a respiratory dysfunction. This demonstrates that mRNA localization to the vicinity of mitochondria plays a critical role in organelle biogenesis.
Figures
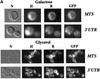
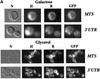
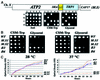
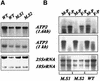
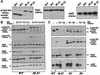
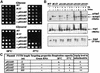
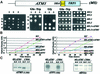
Similar articles
-
Localization of mRNAs coding for mitochondrial proteins in the yeast Saccharomyces cerevisiae.RNA. 2011 Aug;17(8):1551-65. doi: 10.1261/rna.2621111. Epub 2011 Jun 24. RNA. 2011. PMID: 21705432 Free PMC article.
-
In yeast, the 3' untranslated region or the presequence of ATM1 is required for the exclusive localization of its mRNA to the vicinity of mitochondria.Mol Cell Biol. 2000 Nov;20(21):7881-92. doi: 10.1128/MCB.20.21.7881-7892.2000. Mol Cell Biol. 2000. PMID: 11027259 Free PMC article.
-
Why are many mRNAs translated to the vicinity of mitochondria: a role in protein complex assembly?Gene. 2005 Jul 18;354:64-71. doi: 10.1016/j.gene.2005.04.022. Gene. 2005. PMID: 15979254
-
The amino terminus of the yeast F1-ATPase beta-subunit precursor functions as a mitochondrial import signal.J Cell Biol. 1986 Feb;102(2):523-33. doi: 10.1083/jcb.102.2.523. J Cell Biol. 1986. PMID: 2868014 Free PMC article.
-
RNA localization in yeast: moving towards a mechanism.Biol Cell. 2005 Jan;97(1):75-86. doi: 10.1042/BC20040066. Biol Cell. 2005. PMID: 15601259 Review.
Cited by
-
mRNA trafficking in fungi.Mol Genet Genomics. 2007 Oct;278(4):347-59. doi: 10.1007/s00438-007-0271-8. Epub 2007 Sep 1. Mol Genet Genomics. 2007. PMID: 17768642 Review.
-
Differential targeting of VDAC3 mRNA isoforms influences mitochondria morphology.Proc Natl Acad Sci U S A. 2014 Jun 17;111(24):8991-6. doi: 10.1073/pnas.1402588111. Epub 2014 Jun 2. Proc Natl Acad Sci U S A. 2014. PMID: 24889622 Free PMC article.
-
CLUH regulates mitochondrial metabolism by controlling translation and decay of target mRNAs.J Cell Biol. 2017 Mar 6;216(3):675-693. doi: 10.1083/jcb.201607019. Epub 2017 Feb 10. J Cell Biol. 2017. PMID: 28188211 Free PMC article.
-
The role of the 3' untranslated region in mRNA sorting to the vicinity of mitochondria is conserved from yeast to human cells.Mol Biol Cell. 2003 Sep;14(9):3848-56. doi: 10.1091/mbc.e03-02-0074. Epub 2003 Jun 13. Mol Biol Cell. 2003. PMID: 12972568 Free PMC article.
-
Import-associated translational inhibition: novel in vivo evidence for cotranslational protein import into Dictyostelium discoideum mitochondria.Eukaryot Cell. 2006 Aug;5(8):1314-27. doi: 10.1128/EC.00386-05. Eukaryot Cell. 2006. PMID: 16896215 Free PMC article.
References
-
- Ades I.Z. and Butow,R.A. (1980) The transport of proteins into yeast mitochondria. Kinetics and pools. J. Biol. Chem., 255, 9925–9935. - PubMed
-
- Bassell G.J., Oleynikov,Y. and Singer,R.H. (1999) The travels of mRNAs through all cells large and small. FASEB J., 13, 447–454. - PubMed
-
- Beach D.L., Salmon,E.D. and Bloom,K. (1999) Localization and anchoring of mRNA in budding yeast. Curr. Biol., 9, 569–578. - PubMed
-
- Chen L.B. (1989) Fluorescent labeling of mitochondria. Methods Cell Biol., 29, 103–123. - PubMed
Publication types
MeSH terms
Substances
LinkOut - more resources
Full Text Sources
Other Literature Sources
Molecular Biology Databases