Separation and characterization of currents through store-operated CRAC channels and Mg2+-inhibited cation (MIC) channels
- PMID: 11981025
- PMCID: PMC2233817
- DOI: 10.1085/jgp.20028551
Separation and characterization of currents through store-operated CRAC channels and Mg2+-inhibited cation (MIC) channels
Erratum in
- J Gen Physiol 2002 Jun;119(6):613
Abstract
Although store-operated calcium release-activated Ca(2+) (CRAC) channels are highly Ca(2+)-selective under physiological ionic conditions, removal of extracellular divalent cations makes them freely permeable to monovalent cations. Several past studies have concluded that under these conditions CRAC channels conduct Na(+) and Cs(+) with a unitary conductance of approximately 40 pS, and that intracellular Mg(2+) modulates their activity and selectivity. These results have important implications for understanding ion permeation through CRAC channels and for screening potential CRAC channel genes. We find that the observed 40-pS channels are not CRAC channels, but are instead Mg(2+)-inhibited cation (MIC) channels that open as Mg(2+) is washed out of the cytosol. MIC channels differ from CRAC channels in several critical respects. Store depletion does not activate MIC channels, nor does store refilling deactivate them. Unlike CRAC channels, MIC channels are not blocked by SKF 96365, are not potentiated by low doses of 2-APB, and are less sensitive to block by high doses of the drug. By applying 8-10 mM intracellular Mg(2+) to inhibit MIC channels, we examined monovalent permeation through CRAC channels in isolation. A rapid switch from 20 mM Ca(2+) to divalent-free extracellular solution evokes Na(+) current through open CRAC channels (Na(+)-I(CRAC)) that is initially eightfold larger than the preceding Ca(2+) current and declines by approximately 80% over 20 s. Unlike MIC channels, CRAC channels are largely impermeable to Cs(+) (P(Cs)/P(Na) = 0.13 vs. 1.2 for MIC). Neither the decline in Na(+)-I(CRAC) nor its low Cs(+) permeability are affected by intracellular Mg(2+) (90 microM to 10 mM). Single openings of monovalent CRAC channels were not detectable in whole-cell recordings, but a unitary conductance of 0.2 pS was estimated from noise analysis. This new information about the selectivity, conductance, and regulation of CRAC channels forces a revision of the biophysical fingerprint of CRAC channels, and reveals intriguing similarities and differences in permeation mechanisms of voltage-gated and store-operated Ca(2+) channels.
Figures
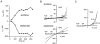
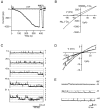
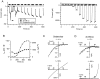
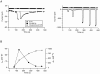
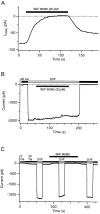
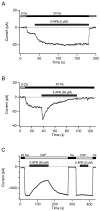
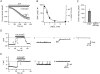
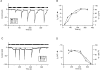
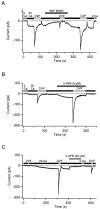
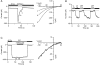
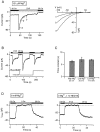
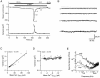
Similar articles
-
Monovalent cation permeability and Ca(2+) block of the store-operated Ca(2+) current I(CRAC )in rat basophilic leukemia cells.Pflugers Arch. 2002 Mar;443(5-6):892-902. doi: 10.1007/s00424-001-0775-8. Epub 2002 Jan 22. Pflugers Arch. 2002. PMID: 11889590
-
Distinct properties of CRAC and MIC channels in RBL cells.J Gen Physiol. 2002 Aug;120(2):221-35. doi: 10.1085/jgp.20028601. J Gen Physiol. 2002. PMID: 12149283 Free PMC article.
-
Regulation of CRAC channel activity by recruitment of silent channels to a high open-probability gating mode.J Gen Physiol. 2006 Sep;128(3):373-86. doi: 10.1085/jgp.200609588. J Gen Physiol. 2006. PMID: 16940559 Free PMC article.
-
Store-Independent Orai Channels Regulated by STIM.In: Kozak JA, Putney JW Jr, editors. Calcium Entry Channels in Non-Excitable Cells. Boca Raton (FL): CRC Press/Taylor & Francis; 2018. Chapter 11. In: Kozak JA, Putney JW Jr, editors. Calcium Entry Channels in Non-Excitable Cells. Boca Raton (FL): CRC Press/Taylor & Francis; 2018. Chapter 11. PMID: 30299650 Free Books & Documents. Review.
-
Permeation through store-operated CRAC channels in divalent-free solution: potential problems and implications for putative CRAC channel genes.Cell Calcium. 2002 Nov-Dec;32(5-6):379-91. doi: 10.1016/s0143416002001914. Cell Calcium. 2002. PMID: 12543097 Review.
Cited by
-
STIM1 and Orai1 mediate CRAC channel activity and are essential for human glioblastoma invasion.Pflugers Arch. 2013 Sep;465(9):1249-60. doi: 10.1007/s00424-013-1254-8. Epub 2013 Mar 21. Pflugers Arch. 2013. PMID: 23515871 Free PMC article.
-
KCa3.1 and TRPM7 channels at the uropod regulate migration of activated human T cells.PLoS One. 2012;7(8):e43859. doi: 10.1371/journal.pone.0043859. Epub 2012 Aug 27. PLoS One. 2012. PMID: 22952790 Free PMC article.
-
How many Orai's does it take to make a CRAC channel?Sci Rep. 2013;3:1961. doi: 10.1038/srep01961. Sci Rep. 2013. PMID: 23743658 Free PMC article.
-
Charge screening by internal pH and polyvalent cations as a mechanism for activation, inhibition, and rundown of TRPM7/MIC channels.J Gen Physiol. 2005 Nov;126(5):499-514. doi: 10.1085/jgp.200509324. J Gen Physiol. 2005. PMID: 16260839 Free PMC article.
-
The Mg2+ and Mg(2+)-nucleotide-regulated channel-kinase TRPM7.Handb Exp Pharmacol. 2007;(179):313-28. doi: 10.1007/978-3-540-34891-7_19. Handb Exp Pharmacol. 2007. PMID: 17217066 Free PMC article. Review.
References
-
- Bakowski, D., and A.B. Parekh. 2002. Monovalent cation permeability and Ca2+ block of the store-operated Ca2+ current ICRAC in rat basophilic leukemia cells. Pflügers Arch. 443:892–902. - PubMed
-
- Braun, F.J., L.M. Broad, D.L. Armstrong, and J.W. Putney, Jr. 2001. Stable activation of single Ca2+ release-activated Ca2+ channels in divalent cation-free solutions. J. Biol. Chem. 276:1063–1070. - PubMed
-
- Broad, L.M., F.J. Braun, J.P. Lievremont, G.S. Bird, T. Kurosaki, and J.W. Putney, Jr. 2001. Role of the phospholipase C-inositol 1,4,5-trisphosphate pathway in calcium release-activated calcium current and capacitative calcium entry. J. Biol. Chem. 276:15945–15952. - PubMed
Publication types
MeSH terms
Substances
Grants and funding
LinkOut - more resources
Full Text Sources
Other Literature Sources
Miscellaneous