A functional role for intra-axonal protein synthesis during axonal regeneration from adult sensory neurons
- PMID: 11717363
- PMCID: PMC6763927
- DOI: 10.1523/JNEUROSCI.21-23-09291.2001
A functional role for intra-axonal protein synthesis during axonal regeneration from adult sensory neurons
Abstract
Although intradendritic protein synthesis has been documented in adult neurons, the question of whether axons actively synthesize proteins remains controversial. Adult sensory neurons that are conditioned by axonal crush can rapidly extend processes in vitro by regulating the translation of existing mRNAs (Twiss et al., 2000). These regenerating processes contain axonal but not dendritic proteins. Here we show that these axonal processes of adult sensory neurons cultured after conditioning injury contain ribosomal proteins, translational initiation factors, and rRNA. Pure preparations of regenerating axons separated from the DRG cell bodies can actively synthesize proteins in vitro and contain ribosome-bound beta-actin and neurofilament mRNAs. Blocking protein synthesis in these regenerating sensory axons causes a rapid retraction of their growth cones when communication with the cell body is blocked by axotomy or colchicine treatment. These findings indicate that axons of adult mammalian neurons can synthesize proteins and suggest that, under some circumstances, intra-axonal translation contributes to structural integrity of the growth cone in regenerating axons. By immunofluorescence, translation factors, ribosomal proteins, and rRNA were also detected in motor axons of ventral spinal roots analyzed after 7 d in vivo after a peripheral axonal crush injury. Thus, adult motor neurons are also likely capable of intra-axonal protein synthesis in vivo after axonal injury.
Figures
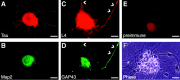
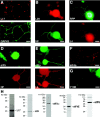
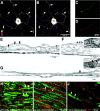
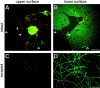
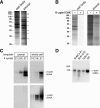
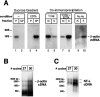
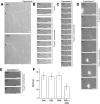
Similar articles
-
mRNAs and Protein Synthetic Machinery Localize into Regenerating Spinal Cord Axons When They Are Provided a Substrate That Supports Growth.J Neurosci. 2015 Jul 15;35(28):10357-70. doi: 10.1523/JNEUROSCI.1249-15.2015. J Neurosci. 2015. PMID: 26180210 Free PMC article.
-
Axonal protein synthesis and degradation are necessary for efficient growth cone regeneration.J Neurosci. 2005 Jan 12;25(2):331-42. doi: 10.1523/JNEUROSCI.3073-04.2005. J Neurosci. 2005. PMID: 15647476 Free PMC article.
-
Guiding adult Mammalian sensory axons during regeneration.J Neuropathol Exp Neurol. 2008 Mar;67(3):212-22. doi: 10.1097/NEN.0b013e3181654972. J Neuropathol Exp Neurol. 2008. PMID: 18344912
-
Translating regeneration: Local protein synthesis in the neuronal injury response.Neurosci Res. 2019 Feb;139:26-36. doi: 10.1016/j.neures.2018.10.003. Epub 2018 Oct 12. Neurosci Res. 2019. PMID: 30321567 Review.
-
New insights into neuronal regeneration: the role of axonal protein synthesis in pathfinding and axonal extension.J Neurotrauma. 2006 Mar-Apr;23(3-4):295-308. doi: 10.1089/neu.2006.23.295. J Neurotrauma. 2006. PMID: 16629617 Review.
Cited by
-
Axonal amphoterin mRNA is regulated by translational control and enhances axon outgrowth.J Neurosci. 2015 Apr 8;35(14):5693-706. doi: 10.1523/JNEUROSCI.3397-14.2015. J Neurosci. 2015. PMID: 25855182 Free PMC article.
-
Anterograde transport and secretion of brain-derived neurotrophic factor along sensory axons promote Schwann cell myelination.J Neurosci. 2007 Jul 11;27(28):7597-603. doi: 10.1523/JNEUROSCI.0563-07.2007. J Neurosci. 2007. PMID: 17626221 Free PMC article.
-
A rapamycin-sensitive signaling pathway is essential for the full expression of persistent pain states.J Neurosci. 2009 Nov 25;29(47):15017-27. doi: 10.1523/JNEUROSCI.3451-09.2009. J Neurosci. 2009. PMID: 19940197 Free PMC article.
-
Transcriptomes of Injured Lamprey Axon Tips: Single-Cell RNA-Seq Suggests Differential Involvement of MAPK Signaling Pathways in Axon Retraction and Regeneration after Spinal Cord Injury.Cells. 2022 Jul 27;11(15):2320. doi: 10.3390/cells11152320. Cells. 2022. PMID: 35954164 Free PMC article.
-
Axonal mRNA in uninjured and regenerating cortical mammalian axons.J Neurosci. 2009 Apr 15;29(15):4697-707. doi: 10.1523/JNEUROSCI.6130-08.2009. J Neurosci. 2009. PMID: 19369540 Free PMC article.
References
-
- Alvarez J, Giuditta A, Koenig E. Protein synthesis in axons and terminals: significance for maintenance, plasticity and regulation of phenotype. With a critique of slow transport theory. Prog Neurobiol. 2000;62:1–62. - PubMed
-
- Baum E, Wormington W. Coordinate expression of ribosomal protein genes during Xenopus development. Dev Biol. 1985;111:488–498. - PubMed
-
- Biberman Y, Meyuhas O. Substitution of just five nucleotides at and around the transcription start site of rat beta-actin promoter is sufficient to render the resulting transcript a subject for translational control. FEBS Lett. 1997;405:333–336. - PubMed
-
- Bittner GD. Long-term survival of anucleate axons and its implications for nerve regeneration. Trends Neurosci. 1991;14:188–193. - PubMed
Publication types
MeSH terms
Substances
LinkOut - more resources
Full Text Sources
Other Literature Sources