Coral larvae increase nitrogen assimilation to stabilize algal symbiosis and combat bleaching under increased temperature
- PMID: 39531470
- PMCID: PMC11556732
- DOI: 10.1371/journal.pbio.3002875
Coral larvae increase nitrogen assimilation to stabilize algal symbiosis and combat bleaching under increased temperature
Abstract
Rising sea surface temperatures are increasingly causing breakdown in the nutritional relationship between corals and algal endosymbionts (Symbiodiniaceae), threatening the basis of coral reef ecosystems and highlighting the critical role of coral reproduction in reef maintenance. The effects of thermal stress on metabolic exchange (i.e., transfer of fixed carbon photosynthates from symbiont to host) during sensitive early life stages, however, remains understudied. We exposed symbiotic Montipora capitata coral larvae in Hawai'i to high temperature (+2.5°C for 3 days), assessed rates of photosynthesis and respiration, and used stable isotope tracing (4 mM 13C sodium bicarbonate; 4.5 h) to quantify metabolite exchange. While larvae did not show any signs of bleaching and did not experience declines in survival and settlement, metabolic depression was significant under high temperature, indicated by a 19% reduction in respiration rates, but with no change in photosynthesis. Larvae exposed to high temperature showed evidence for maintained translocation of a major photosynthate, glucose, from the symbiont, but there was reduced metabolism of glucose through central carbon metabolism (i.e., glycolysis). The larval host invested in nitrogen cycling by increasing ammonium assimilation, urea metabolism, and sequestration of nitrogen into dipeptides, a mechanism that may support the maintenance of glucose translocation under thermal stress. Host nitrogen assimilation via dipeptide synthesis appears to be used for nitrogen limitation to the Symbiodiniaceae, and we hypothesize that nitrogen limitation contributes to retention of fixed carbon by favoring photosynthate translocation to the host. Collectively, our findings indicate that although these larvae are susceptible to metabolic stress under high temperature, diverting energy to nitrogen assimilation to maintain symbiont population density, photosynthesis, and carbon translocation may allow larvae to avoid bleaching and highlights potential life stage specific metabolic responses to stress.
Copyright: © 2024 Huffmyer et al. This is an open access article distributed under the terms of the Creative Commons Attribution License, which permits unrestricted use, distribution, and reproduction in any medium, provided the original author and source are credited.
Conflict of interest statement
The authors have declared that no competing interests exist.
Figures
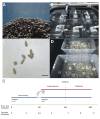
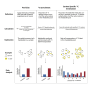
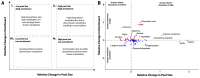
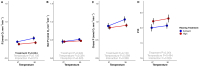
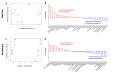
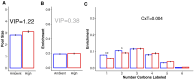
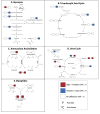
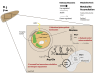
Comment in
-
Youthful insight: Nitrogen sequestration in larvae provides clues to coral bleaching.PLoS Biol. 2024 Nov 13;22(11):e3002890. doi: 10.1371/journal.pbio.3002890. eCollection 2024 Nov. PLoS Biol. 2024. PMID: 39536009 Free PMC article.
Similar articles
-
Symbiodiniaceae algal symbionts of Pocillopora damicornis larvae provide more carbon to their coral host under elevated levels of acidification and temperature.Commun Biol. 2024 Nov 18;7(1):1528. doi: 10.1038/s42003-024-07203-4. Commun Biol. 2024. PMID: 39558079 Free PMC article.
-
Climate change promotes parasitism in a coral symbiosis.ISME J. 2018 Mar;12(3):921-930. doi: 10.1038/s41396-018-0046-8. Epub 2018 Jan 29. ISME J. 2018. PMID: 29379177 Free PMC article.
-
Coral species-specific loss and physiological legacy effects are elicited by an extended marine heatwave.J Exp Biol. 2024 Jun 1;227(11):jeb246812. doi: 10.1242/jeb.246812. Epub 2024 Jun 17. J Exp Biol. 2024. PMID: 38774956
-
Nutrient Availability and Metabolism Affect the Stability of Coral-Symbiodiniaceae Symbioses.Trends Microbiol. 2019 Aug;27(8):678-689. doi: 10.1016/j.tim.2019.03.004. Epub 2019 Apr 12. Trends Microbiol. 2019. PMID: 30987816 Review.
-
Coral bleaching--capacity for acclimatization and adaptation.Adv Mar Biol. 2003;46:183-223. doi: 10.1016/s0065-2881(03)46004-5. Adv Mar Biol. 2003. PMID: 14601413 Review.
Cited by
-
Youthful insight: Nitrogen sequestration in larvae provides clues to coral bleaching.PLoS Biol. 2024 Nov 13;22(11):e3002890. doi: 10.1371/journal.pbio.3002890. eCollection 2024 Nov. PLoS Biol. 2024. PMID: 39536009 Free PMC article.
References
-
- Muscatine L, Porter JW. Reef Corals: Mutualistic Symbioses Adapted to Nutrient-Poor Environments. Bioscience. 1977;27:454–460. doi: 10.2307/1297526 - DOI
-
- Falkowski PG, Dubinsky Z, Muscatine L, Porter JW. Light and the bioenergetics of a symbiotic coral. Bioscience. 2006;34:705–709. doi: 10.2307/1309663 - DOI
MeSH terms
Substances
Grants and funding
LinkOut - more resources
Full Text Sources