The dithiol mechanism of class I glutaredoxins promotes specificity for glutathione as a reducing agent
- PMID: 39488995
- PMCID: PMC11567954
- DOI: 10.1016/j.redox.2024.103410
The dithiol mechanism of class I glutaredoxins promotes specificity for glutathione as a reducing agent
Abstract
Class I glutaredoxins reversibly reduce glutathione- and nonglutathione disulfides with the help of reduced glutathione (GSH) using either a monothiol mechanism or a dithiol mechanism. The monothiol mechanism exclusively involves a single glutathionylated active-site cysteinyl residue, whereas the dithiol mechanism requires the additional formation of an intramolecular disulfide bond between the active-site cysteinyl residue and a resolving cysteinyl residue. While the oxidation of glutaredoxins by glutathione disulfide substrates has been extensively characterized, the enzyme-substrate interactions for the reduction of S-glutathionylated glutaredoxins or intramolecular glutaredoxin disulfides are still poorly characterized. Here we compared the thiol-specificity for the reduction of S-glutathionylated glutaredoxins and the intramolecular glutaredoxin disulfide. We show that S-glutathionylated glutaredoxins rapidly react with a plethora of thiols and that the 2nd glutathione-interaction site of class I glutaredoxins lacks specificity for GSH as a reducing agent. In contrast, the slower reduction of the partially strained intramolecular glutaredoxin disulfide involves specific interactions with both carboxylate groups of GSH at the 1st glutathione-interaction site. Thus, the dithiol mechanism of class I glutaredoxins promotes specificity for GSH as a reducing agent, which might explain the prevalence of dithiol glutaredoxins in pro- and eukaryotes.
Keywords: Disulfide; Dithiol; Enzyme mechanism; Glutaredoxin; Glutathione; Redox catalysis; Stopped-flow kinetics.
Copyright © 2024 The Authors. Published by Elsevier B.V. All rights reserved.
Conflict of interest statement
Declaration of competing interest The authors declare no competing interests. There are no financial/personal interests or beliefs that could affect our objectivity or result in a potential conflict.
Figures
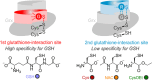
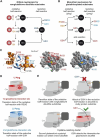
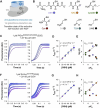
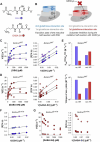
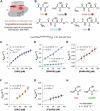
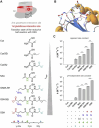
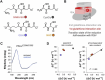
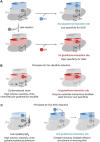
Similar articles
-
Depressing time: Waiting, melancholia, and the psychoanalytic practice of care.In: Kirtsoglou E, Simpson B, editors. The Time of Anthropology: Studies of Contemporary Chronopolitics. Abingdon: Routledge; 2020. Chapter 5. In: Kirtsoglou E, Simpson B, editors. The Time of Anthropology: Studies of Contemporary Chronopolitics. Abingdon: Routledge; 2020. Chapter 5. PMID: 36137063 Free Books & Documents. Review.
-
Comparison of Two Modern Survival Prediction Tools, SORG-MLA and METSSS, in Patients With Symptomatic Long-bone Metastases Who Underwent Local Treatment With Surgery Followed by Radiotherapy and With Radiotherapy Alone.Clin Orthop Relat Res. 2024 Dec 1;482(12):2193-2208. doi: 10.1097/CORR.0000000000003185. Epub 2024 Jul 23. Clin Orthop Relat Res. 2024. PMID: 39051924
-
Molecular basis for the enzymatic inactivity of class III glutaredoxin ROXY9 on standard glutathionylated substrates.Nat Commun. 2025 Jan 11;16(1):589. doi: 10.1038/s41467-024-55532-z. Nat Commun. 2025. PMID: 39799154 Free PMC article.
-
Antioxidants for female subfertility.Cochrane Database Syst Rev. 2020 Aug 27;8(8):CD007807. doi: 10.1002/14651858.CD007807.pub4. Cochrane Database Syst Rev. 2020. PMID: 32851663 Free PMC article.
-
Antioxidants for female subfertility.Cochrane Database Syst Rev. 2017 Jul 28;7(7):CD007807. doi: 10.1002/14651858.CD007807.pub3. Cochrane Database Syst Rev. 2017. Update in: Cochrane Database Syst Rev. 2020 Aug 27;8:CD007807. doi: 10.1002/14651858.CD007807.pub4. PMID: 28752910 Free PMC article. Updated. Review.
References
-
- Holmgren A. Thioredoxin. Annu. Rev. Biochem. 1985;54:237–271. - PubMed
-
- Jensen K.S., Hansen R.E., Winther J.R. Kinetic and thermodynamic aspects of cellular thiol-disulfide redox regulation. Antioxidants Redox Signal. 2009;11:1047–1058. - PubMed
-
- Deponte M. Glutathione catalysis and the reaction mechanisms of glutathione-dependent enzymes. Biochim. Biophys. Acta. 2013;1830:3217–3266. - PubMed
MeSH terms
Substances
LinkOut - more resources
Full Text Sources