Structural and biochemical analysis of ligand binding in yeast Niemann-Pick type C1-related protein
- PMID: 39455279
- PMCID: PMC11512107
- DOI: 10.26508/lsa.202402990
Structural and biochemical analysis of ligand binding in yeast Niemann-Pick type C1-related protein
Abstract
In eukaryotes, integration of sterols into the vacuolar/lysosomal membrane is critically dependent on the Niemann-Pick type C (NPC) system. The system consists of an integral membrane protein, called NCR1 in yeast, and NPC2, a luminal soluble protein that transfers sterols to the N-terminal domain (NTD) of NCR1 before membrane integration. Both proteins have been implicated in sterol homeostasis of yeast and humans. Here, we investigate sterol and lipid binding of the NCR1/NPC2 transport system and determine crystal structures of the sterol binding NTD. The NTD binds both ergosterol and cholesterol, with nearly identical conformations of the binding pocket. Apart from sterols, the NTD can also bind fluorescent analogs of phosphatidylinositol, phosphatidylcholine, and phosphatidylserine, as well as sphingosine and ceramide. We confirm the multi-lipid scope of the NCR1/NPC2 system using photo-crosslinkable and clickable lipid analogs, namely, pac-cholesterol, pac-sphingosine, and pac-ceramide. Finally, we reconstitute the transfer of pac-sphingosine from NPC2 to the NTD in vitro. Collectively, our results support that the yeast NPC system can work as versatile machinery for vacuolar homeostasis of structurally diverse lipids, besides ergosterol.
© 2024 Nel et al.
Conflict of interest statement
The authors declare that they have no conflict of interest.
Figures
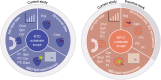
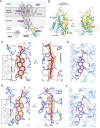
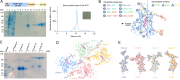
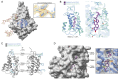
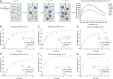
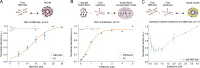
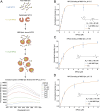
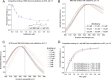
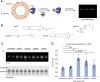
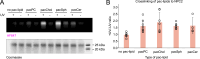
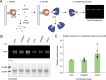
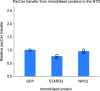
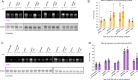
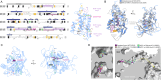
Similar articles
-
Automated quantification of vacuole fusion and lipophagy in Saccharomyces cerevisiae from fluorescence and cryo-soft X-ray microscopy data using deep learning.Autophagy. 2024 Apr;20(4):902-922. doi: 10.1080/15548627.2023.2270378. Epub 2023 Oct 31. Autophagy. 2024. PMID: 37908116 Free PMC article.
-
Enabling Systemic Identification and Functionality Profiling for Cdc42 Homeostatic Modulators.bioRxiv [Preprint]. 2024 Jan 8:2024.01.05.574351. doi: 10.1101/2024.01.05.574351. bioRxiv. 2024. Update in: Commun Chem. 2024 Nov 19;7(1):271. doi: 10.1038/s42004-024-01352-7. PMID: 38260445 Free PMC article. Updated. Preprint.
-
Comparison of Two Modern Survival Prediction Tools, SORG-MLA and METSSS, in Patients With Symptomatic Long-bone Metastases Who Underwent Local Treatment With Surgery Followed by Radiotherapy and With Radiotherapy Alone.Clin Orthop Relat Res. 2024 Dec 1;482(12):2193-2208. doi: 10.1097/CORR.0000000000003185. Epub 2024 Jul 23. Clin Orthop Relat Res. 2024. PMID: 39051924
-
Depressing time: Waiting, melancholia, and the psychoanalytic practice of care.In: Kirtsoglou E, Simpson B, editors. The Time of Anthropology: Studies of Contemporary Chronopolitics. Abingdon: Routledge; 2020. Chapter 5. In: Kirtsoglou E, Simpson B, editors. The Time of Anthropology: Studies of Contemporary Chronopolitics. Abingdon: Routledge; 2020. Chapter 5. PMID: 36137063 Free Books & Documents. Review.
-
The effectiveness of abstinence-based and harm reduction-based interventions in reducing problematic substance use in adults who are experiencing homelessness in high income countries: A systematic review and meta-analysis: A systematic review.Campbell Syst Rev. 2024 Apr 21;20(2):e1396. doi: 10.1002/cl2.1396. eCollection 2024 Jun. Campbell Syst Rev. 2024. PMID: 38645303 Free PMC article. Review.
References
MeSH terms
Substances
Associated data
- Actions
- Actions
- Actions
- Actions
- Actions
- Actions
- Actions
- Actions
LinkOut - more resources
Full Text Sources